Introduction
With the rising demand of food security due to the ever-increasing population growth coupled with a looming threat of climate change (Haque et al. 2018; United Nations 2019), the urgency to develop reliable and efficient methods to secure a steady and sufficient nutrition to the global population is higher than ever. Hence, the role and application of plant biotechnology to engineer plants to suit global agricultural demands are now indispensable. Plant biotechnology (PBT), in essence, comprises the set of scientific methods and techniques used to identify and manipulate plant genes in order to develop desired traits or specific products in plants (Kalia, 2018). By using the methods available in PBT, beneficial traits of crops can be expressed and amplified, while undesirable traits and components such as allergens in rice, peanuts, or soybeans can be eliminated (Fuchs and Mackey, 2003; Barh and Azevedo, 2018).
The emergence of Sequence-Specific Nucleases (SSNs) such as Zinc Finger Nucleases (ZFNs), Transcriptional Activator-like Effector Nucleases (TALENs), and the more recently developed Clustered Regularly Interspaced Short Palindromic Repeats (CRISPR)/CRISPR-associated protein 9 (Cas9) are among the advanced methods that allowed a less sporadic and more precise means of genetic modifications in plants (Baltes et al., 2014; Fauser et al., 2014; Endo et al., 2016; Li et al., 2016; Sun et al., 2016a; Sun et al., 2016b). A detailed review on the comparison between the abovementioned three methods was done by Sun et al. (2016a). The CRISPR/Cas9 system is generally preferred over the other methods because of its precision, efficiency, simplicity, and cost-effectiveness. Hence, it has gained attention in the genome editing community (Haque et al., 2018; Wang et al., 2018). Since the discovery of the first CRISPR locus by Ishino et al. (1987) and the pioneering extensive study on the CRISPR/Cas system by Jansen et al. (2002), the technology has been further studied for genome editing of various organisms.
In essence, CRISPR/Cas9 technology exploits the adaptive immunity system of the bacteria Streptococcus pyogenes in DNA repair to modify the genetic sequences or even edit the genome of the targeted organism. This is achieved by constructing a single guide RNA (sgRNA) specific to the target DNA sequence, which forms a complex with the Cas9 protein, thereby initiating specific doublestranded breaks in the target DNA, as shown in Fig. 1 (Costa et al., 2017). The double-stranded breaks enable further gene editing as shown in Figure 1D and Figure 1E. Many studies have been conducted to describe the mechanism of the CRISPR/Cas9 system, as were extensively reviewed by researchers (Sander and Joung, 2014; Westra et al., 2014; Bortesi and Fischer, 2015; Ma et al., 2015; Ma et al., 2016; Musunuru, 2017; Adhikari and Poudel, 2020). Hence, to follow suit, this review discusses 1) the recent advances in utilizing the CRISPR/Cas9 system in a diverse range of plant species for crop enhancement and facilitate plant cell imaging, 2) the current challenges faced regarding the delivery methods of CRISPR/Cas9 reagents into plant cells, and 3) the regulatory systems of gene edited crops compared to those for genetically modified organisms (GMOs).
Fig. 1
Mechanism of CRISPR/Cas9 gene editing. A) The constructed target-specific single guide RNA (sgRNA) forms a complex with the Cas9 protein; B) The CRISPR/Cas9 complex binds to the target DNA; C) The CRISPR/Cas9 cleaves the target DNA at specific sequences, leading to further gene editing; D) Gene knock-in through homology-directed repair (HDR); E) Gene knock-out through non-homologous end joining (NHEJ)
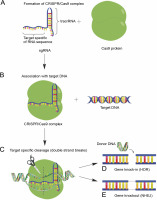
Applications of CRISPR/Cas9 genome editing in plant species
Certain phenotypes or traits that are expressed by plants, or in this case crops, can be tweaked and adjusted through the manipulation of their genes. In doing so, the expected outcome would be to produce an enhanced version of the crop, which can be beneficial to the general population from certain aspects. The precision of CRISPR/Cas9 technology ensures a highly reliable method in genome editing that does not randomly produce unforeseen alterations elsewhere in the genome (Schiml et al., 2016). The efforts in trying to apply CRISPR/Cas9 genome editing in plants have been widespread since the discovery of the technology. Prior to applying the genome editing technology to crops, much of the research was conducted on Arabidopsis thaliana as a model plant organism because of its convenience and usefulness in genetic experiments (Koornneef and Meinke, 2010; Lee et al., 2018).
For example, A. thaliana was used as a model plant in implementing a sequential transformation method, which improved CRISPR gene targeting (Miki et al., 2018). The efficiency of pKAMA-ITACHI Red vector in CRISPR/Cas9 was also first investigated in A. thaliana when a study involving genes such as PDS3, AG, and DUO1, was conducted by Tsutsui and Higashiyama (2017). After the initial validation on A. thaliana, the potentials of the technology are being further explored in other plant species. Some examples of plants and crops that have been successfully manipulated using CRISPR/Cas9 technology during the recent years are outlined in Table 1.
Table 1
Examples of successful genome editing of plant species
Plant | Gene(s) targeted | Traits | Method | References |
---|---|---|---|---|
Apple | MdDIPM4 | disease resistance | gene inactivation | Pompili et al. 2020 |
Maize | ZmPHYC1 ZmPHYC2 | flowering time/plant height | gene knockout & overexpression | Li et al. 2020 |
Muskmelon | CmPDS | albinism (CRISPR trial) | gene knockout | Hooghvorst et al. 2019 |
Oil palm | EgIFR EgMT | disease resistance | base editing | Budiani et al. 2018 |
Oilseed rape | BnALS1 | herbicide resistance | base editing | Wu et al. 2020 |
BnaSDG8.A BnaSDG8.B | plant flowering | gene knockout/down | Jiang et al. 2018 | |
Rice | Os8N3 OsProDH OsGS3 OsNAC45 | disease resistance thermotolerance grain length salt tolerance | gene knockou gene knockout & overexpression site directed mutagenesis gene knockout & overexpression | Kim et al. 2019 Guo et al. 2020 Usman et al. 2021 X. Zhang et al. 2020 |
Soybean | GmPRR37 GmFT2a/5a | flowering time & regional adaptability | site directed mutagenesis | Cai et al., 2018; Cai et al., 2020; Wang et al., 2020 |
GmF3H1 GmF3H2 GmF3FNSII-1 | disease resistance | multiplex gene knockout | P. Zhang et al., 2020 | |
Tobacco | NtHL1 | hybrid lethality | frameshift mutation | Ma et al., 2020 |
Watermelon | ClPDS | albinism (CRISPR trial) | gene knockout | Tian et al., 2017 |
Improvement on quality of crops
Rice
One of the more impactful applications of CRISPR/Cas9 technology from the sustainability aspect is the ability of the genome editing tool to enhance the quality of agricultural products. Rice, a major food source for the global population (Fukagawa and Ziska, 2019), was first successfully manipulated using the CRISPR/Cas9 technology by Miao et al. (2013), who demonstrated the possibility of applying the system for targeted mutations in rice. Since this finding, many efforts have been channeled to elucidate the functions of individual genes and observe the effect of gene alterations in rice in the hope to apply the findings practically. An example would be a study by Guo et al. (2020), who used CRISPR/Cas9 to both induce overexpression and knockout the OsProDH gene in rice. The OsProDH gene encodes for a mitochondrial enzyme, proline dehydrogenase, which is responsible for the degradation of proline in rice. Proline plays a significant role in protecting plants from various biotic and abiotic stresses by inducing diverse physiological responses of the plants and by scavenging reactive oxygen species (ROS) (Hayat et al., 2012). It was found that mutation in OsProDH in rice resulted in the accumulation of proline, which in turn led to lower levels of ROS (Guo et al., 2020). Hence, by manipulating the OsProDH gene and subsequently the metabolism of proline, higher thermotolerance could be conferred onto rice (Guo et al., 2020).
On the other hand, salt-tolerant rice can be potentially achieved through the manipulation of the OsNAC45 gene (Yu et al., 2018; Zhang et al., 2020b). Through the regulation of several other plant stress response genes (OsCYP89G1, OsDREB1F, OsEREBP2, OsERF104, OsPM1, OsSAMDC2, OsSIK1 ), OsNAC45 may be significant in regulating abscisic acid signal responses in rice, which could be the key to produce rice with increased salt tolerance (Zhang et al., 2020b). In another study, CRISPR/Cas9 was used to elucidate the role of polygalacturonase in regulating the cell wall immune response through the gene OsPG1 (Cao et al., 2021). This not only deepens understanding of the role of cell wall integrity in plant immune response but also highlights the potential to exploit the cell wall physiology in conferring bacterial resistance. Other research studies used CRISPR/Cas9 in a similar manner with the aim of producing an observable effect either through gene overexpression or gene knockout in rice. Through these methods, various genes have been identified and successfully manipulated, for example, genes responsible for traits such as pigment (anthocyanin) content (Zheng et al., 2019; Hu et al., 2020), resistance to disease (bacterial blight and blast disease) (Zhou et al., 2015; Wang et al., 2016; Kim et al., 2019), and grain length (Li et al., 2020a; Usman et al., 2021). These findings prove that CRISPR/Cas9 technology is undoubtedly effective in manipulating traits in rice, and it is expected that these methods can be applied to generate more resilient, robust, and nutritious rice, which can drive global sustainability.
Soybean
CRISPR/Cas9-based mutagenesis has also been successfully performed on other impactful plant species with significant mutation efficiency. The first application of CRISPR/Cas9 gene editing in soybean was conducted by Jacobs et al. (2015) where gene knockout was performed on the green fluorescent protein (GFP) gene. This pioneer work kickstarted numerous efforts in applying CRISPR/Cas9 gene editing in soybean. Han et al. (2019) utilized CRISPR/Cas9 to induce a targeted mutation in the E1 gene in controlling soybean flowering and found that the truncation of the E1 protein prevented the inhibition of the GmFT2a/5a gene, increased its expression, and led to an earlier flowering time under long-day (LD) conditions. This transformation led to the development of a photo-insensitive soybean variant, which is potentially suitable for the introduction of soybean in higher latitudes (Han et al., 2019).
Similarly, a study conducted by Cai et al. (2020) demonstrated the role of the GmFT2a/5a gene in soybean in regulating flowering times and yield under different photoperiods by comparing double-knockouts and overexpression of the gene using CRISPR/Cas9 technology. These findings collectively established the involvement of certain genes in soybean that may contribute to its adaptability in different environments and conditions. Furthermore, the GmFT2a/5a double-knockout mutants were found to produce a significantly higher amount of pods and seeds per plants as compared to the wild-type plant, despite having a longer flowering time (Cai et al., 2020). In addition, the overexpression of GmPRR37 was found to lengthen flowering time under LD conditions and was involved in downregulating the aforementioned GmFT2a/5a, which promotes flowering, and in upregulating GmFT1a that inhibits flowering, thereby contributing to the regional adaptability of soybean (Wang et al., 2020). From these results, soybean variants with a higher productivity can be bred and adapted to a more diverse environment. Triple knockouts of GmF3H1, GmF3H2, and GmFNSII-1 were effectively performed using a multiplex CRISPR/Cas9 system in soybean and resulted in an increase in isoflavone content within the plants that at the same time conferred enhanced resistance to the soybean mosaic virus (SMV) (Zhang et al., 2020a). Several genome edits in soybean were successfully inherited to subsequent generations (Han et al., 2019; Zhang et al., 2020a), indicating that selective breeding of CRISPR/Cas9-edited soybean could potentially generate beneficial novel crop variants. However, the inheritance of CRISPR/Cas9 mutations requires further studies as the efficiency of its occurrence is still rather sporadic.
Oilseed rape
Oilseed rape (Brassica napus ), also known as rapeseed, is another impactful crop that is notable for the production of edible oils (Cartea et al., 2019). The success in CRISPR/Cas9-mediated mutagenesis of rapeseed was first reported by Yang et al. (2017) where 12 genes from four gene families (BnaA9.RGA, BnaC9.RGA, BnaA6.RGA, and BnaC7.RGA from the BnaRGA family; BnaA9.FUL, BnaC2.FUL, and BnaC7FUL from the BnaFUL family; and BnaA2.DA2.1, BnaA2.DA2.2, BnaC6.DA2, BnaC5.DA1, and BnaA6.DA1 from the BnaDA2 and BnaDA1 families) were tested in the study. Subsequently, stable inheritance of the induced mutations by the following progeny was observed in the study, indicating the effectiveness of CRISPR/Cas9 in producing an enhanced variant of oilseed rape (Yang et al., 2017). Following this study, Jiang et al. (2018) successfully identified the role of the BnaSDG8.A and BnaSGD8.C genes in promoting the expression of histone 3 lysine 36 (H3K36) methyltransferase, consequently influencing floral transition in oilseed rape as well as mutating the aforementioned genes to produce an early flowering phenotype. In addition, silencing the BnSFAR4 and BnSFAR5 genes in CRISPR/Cas9-mediated double gene knockout could increase the seed oil content (SOC) in oilseed rape without affecting seed germination, vigor, and oil mobilization, as demonstrated by Karunarathna et al. (2020). In another study, CRISPR/Cas9-mediated cytosine base-editing (CBE) was used in mutating the BnALS1 gene by introducing a C to T conversion at the specific region (Wu et al., 2020). This mutation produced a mutant oilseed rape that could resist tribenuron-methyl, a herbicide commonly used against weeds (Wu et al., 2020). Hence, the development of herbicide resistance in oilseed rape will help farmers in weed management. Taken together, these findings help to drive the productivity and to simplify the management of oilseed rape crop.
Other crop species
Currently, CRISPR/Cas9 genome editing has been demonstrated to be successful on a number of influential crops such as maize (Liu et al., 2020; Li et al., 2020b), wheat (Hayta et al., 2019; Liu et al., 2020), and apples (Pompili et al., 2020), with a relatively high transformation efficiency (Haque et al., 2018; Adhikari and Poudel, 2020). The sequencing of novel plant genomes had widened the applications of CRISPR/Cas9 genome editing in testing higher number of genes in various plant species. CRISPR/Cas9 was recently reported to be effective in knocking out the phytoene desaturase gene in muskmelon (CmPDS), which is the first reported study to apply CRISPR/Cas9 genome editing on the species (Hooghvorst et al., 2019). The same PDS gene was also successfully knocked out to produce an albino phenotype in CRISPR/Cas9 genome editing pioneering studies on watermelon and apples (Nishitani et al., 2016; Tian et al., 2017). However, the rate of inheritance by the subsequent generations of transgenic plants could not be investigated through PDS gene knockout as the albino variants had low in vitro survival rates (Hooghvorst et al., 2019); hence, other genes should be targeted to determine the rate of inheritance of mutations in these plant species.
Targeted mutagenesis in sweet orange was achieved by Jia and Nian (2014), where a novel tool for delivering the CRISPR/Cas9 reagents was developed for citrus plants through the Xcc-facilitated agroinfiltration, and involved the use of Xanthomonas citri subsp. citri (Xcc) to infect the citrus plant. Knockout of the CsWRKY22 gene in Wanjincheng orange using CRISPR/Cas9 genome editing exhibited enhanced resistance toward citrus canker, a destructive disease in citrus plants caused by Xcc, thereby further establishing the efficacy of CRISPR/Cas9 technology in citrus (Wang et al., 2019). Similar enhancement of disease resistance was observed in apples where the successful CRISPR/Cas9-mediated gene knockout of MdDIPM4 conferred increased resistance to Erwinia amylovora, a bacterium that causes fire blight disease in apples (Pompili et al., 2020). Pompili et al. (2020) could successfully clear CRISPR/Cas9 reagents from the genome by using T-DNA removal, which reduced the chances of occurrence of unnecessary or off-target mutations. As a conclusion, CRISPR/Cas9 technology can be applied to a diverse range of plant species and can produce a multitude of effects expressed by the plants. It is expected that the benefits of CRISPR/Cas9-edited crops and products would be able to reach the consumers. This, however, comes with its own set of challenges, one of which will be discussed in the later sections.
Live cell CRISPR imaging
Conventional cellular imaging methods applied in subnuclear dynamics studies such as fluorescence in situ hybridization (FISH) (Langer-Safer et al., 1982; Schwarzacher and Heslop-Harrison, 1994; Wu et al., 2019) are limited by the need of cellular fixation and the heat denaturation step that influence chromatin structure and organization, consequently impeding temporal studies in plant cells (Kozubek et al., 2000; Boettiger et al., 2016; Dreissig et al., 2017). Live cell imaging in plants allows spatiotemporal organization of chromatin to be studied in greater detail, which may potentially deepen the understanding of various gene expression patterns. Novel approaches in live cellular imaging tend to use Zinc Fingers (ZFs) or Transcription Activator-like Effectors (TALEs), which are proteins that can be programmed to bind to specific DNA sequences (Qin et al., 2017; Wu et al., 2019). Even though ZFs and TALEs are more flexible than FISH, there are technical challenges that one has to face as complicated processes are involved in constructing a large array of ZFs and TALEs proteins (Qin et al., 2017) and in constructing their expression vectors capable of targeting multiple DNA sequences (Wu et al., 2019). The necessity of re-engineering TALEs in targeting to a new gene sequence is also time-consuming and labor-intensive (Khosravi et al., 2020).
In view of the limitations of ZFs, TALEs, and FISH, researchers are utilizing the CRISPR/Cas system to achieve a live cell imaging method with greater flexibility and to overcome the limitations of visualizing non-repetitive regions (Dreissig et al., 2017). In this most recent approach, the nuclease activity-deficient dead Cas9 (dCas9), which was shown to possess specific DNA binding ability without DNA alterations (Qi et al., 2013; Dominguez et al., 2016), is combined with a fluorescence protein (FP) to visualize telomeric repeats in live leaf cells of Nicotiana benthamiana. The study proved the usefulness of this method to observe DNA-protein interactions in live plant cells (Dreissig et al., 2017). Telomere repeats in Nicotiana tabacum were also successfully labeled by transiently expressing dCas9-FP, mediated by an Agrobacterium vector (Fujimoto and Matsunaga, 2017). A protocol on conducting live plant cell imaging using CRISPR/Cas9 from S. pyogenes and Staphylococcus aureus was developed by Khosravi et al. (2020), where a telomere-specific guide RNA was used to target the telomeric sequences in N. benthamiana. Through these initial findings, the CRISPR/Cas9 imaging system shows potential for further development in visualizing gene sequences with low repetition or low abundance. Simple and reliable imaging of chromatin spatiotemporal organization would also ease further research on gene expression at various stages of the plant cell cycle. dCas9 can also be applied in gene expression inhibition, transcriptional regulation, gene promoter activation and for monitoring spatiotemporal patterns of gene expression in plants (Bikard and Marraffini, 2013; Yang, 2015; Arora and Narula, 2017). This shows that studies on a single system may potentially yield outcomes that can be beneficial and applied to multiple areas of interest. The potential of the CRISPR/Cas9 system has barely been explored, and more is yet to come.
Challenges in applying CRISPR/Cas9 technology in plants
As a relatively novel toolbox for genome editing, there are certainly some obstacles to be resolved when trying to apply CRISPR/Cas9 technology in plants. First, before any manipulation can be performed on the genome, the specific gene responsible for the intended function must be identified to enable precise editing. Despite the efforts conducted to sequence the genomes of many relevant plant species, there is still insufficient knowledge on the function of sequenced genes within the plants’ genome, which impedes efforts in precision editing to produce intended effects (Haque et al., 2018; Adhikari and Poudel, 2020). Fortunately, by conducting Genome-Wide Association Studies (GWAS), gene functions can be effectively predicted with accuracy, which can drive further research on necessary manipulations in plants. For instance, Zheng et al. (2019) discovered the genes OsC1 and OsRb that are involved in regulating anthocyanins in rice leaf. This enabled Hu et al. (2020) to further use the CRISPR/Cas9 technology in manipulating anthocyanin levels in rice. A similar approach was also undertaken to study the RDP1 gene of A. thaliana (Tsuchimatsu et al., 2020). Just as how GWAS can propel CRISPR/Cas9 plant editing, CRISPR/Cas9 technology is also used as an alternative method for cross population validation (Alseekh et al., 2021), such as to validate GWAS findings in rice (Oryza sativa) (Lu et al., 2017; Meng et al., 2017) and maize (Zea mays) (Liu et al., 2020). This provides an insight into the importance of establishing the causal relationships and interactions between genes that can further drive the development of CRISPR/Cas9 technology in plants (Yin et al., 2017).
Delivery and disposal of CRISPR/Cas9 reagents in plants
The delivery process of the necessary CRISPR/Cas9 components into intended cells remains a challenge to its application in plant and animal cells alike, especially in an in vivo setting (Li et al., 2015). Agrobacterium-mediated delivery using A. tumefaciens or A. rhizogenes is a commonly used method for plant transformation in various species (Ron et al., 2014; Mikami et al., 2015; Budiani et al., 2018; Hooghvorst et al., 2019; Mao et al., 2019; Pompili et al., 2020; Li et al., 2020b). Despite its popularity, there is still a degree of uncertainty when utilizing this method as its success depends on the choice of the plasmid and the cultivar used (Mangena et al., 2017). Various studies have reported that the A. rhizogenes-mediated transformation system could have been the cause of low transformation efficiency observed in soybean (Li et al., 2019; Bai et al., 2020; Zhang et al., 2020a), rice (Butt et al., 2017; Usman et al., 2021), and tomato (Ron et al., 2014) genome editing. Varying culture conditions can also influence the infection and regeneration rates of the Agrobacterium-infected explants, which affects the reproducibility of the results obtained (Hamada et al., 2018) as observed in soybean (Li et al., 2017; Hada, 2018; Mangena, 2018), clover (Trifolium subterraneum L.) (Rojo, 2021), and cassava (Nyaboga et al., 2015). This further showed inconsistencies observed in transformation efficiency through Agrobacteriummediated delivery. In addition, while a high degree of success was observed in A. thaliana, the feasibility of Agrobacterium-mediated transformation in other plant species such as soybean (Mangena et al., 2017), melon (Hooghvorst et al., 2019), and wheat (Zhang et al., 2018) is still questionable, where the regeneration of transgenic plants would require the use of explant-derived calluses (Mao et al., 2019). Hence, further studies are required to enhance the Agrobacterium-mediated delivery method to increase its transformation efficiency, effectiveness in diverse plant species, and its success for in planta transformation.
An alternative to the Agrobacterium-mediated delivery system is biolistic delivery (Carter and Shieh, 2015). Biolistic delivery is the direct delivery of DNA material into plant cells, where DNA is coated onto heavy metal particles such as gold or tungsten (Baltes et al., 2017). As the DNA-coated metal particles penetrate and get trapped inside plant cells, DNA can dissociate from the particles and become integrated into the host genome (Baltes et al., 2017). Although recent success in inducing in planta genome manipulation was observed in wheat (Triticum aestivum L.), the mutation efficiency that was reported using the biolistic method remains very low, less than 6% of samples being mutated and less than 2% of samples with the mutations inherited (Hamada et al., 2018).
Another alternative involves the use of viral vectors as a delivery system for the CRISPR/Cas9 components. A study by Ma et al. (2020) utilizing the sonchus yellow net rhabdovirus (SYNV) to infect tobacco plants reported relatively high mutation efficiency with minimal costs, but the disadvantage of using viral vectors lies in the range of infectivity of the proposed virus. Nonetheless, reverse genetic tools can aid in expanding the range of infectivity for other rhabdoviruses (Ma et al., 2020). Hence, in planta genome editing using CRISPR/Cas9 is currently limited by the availability of effective delivery systems, and further studies and development of conventional and novel delivery methods would contribute to efficient research of CRISPR/Cas9 in plants.
CRISPR/Cas9-edited crop regulation
The ultimate goal of developing novel methods and innovations in applying the CRISPR/Cas9 technology in PBT is to enhance the quality of life of consumers through the production of transgenic plants or crops. Gene-edited organisms such as the ones edited using CRISPR/Cas9 technology involve mutagenesis of their genomes through either deletions, substitutions, or insertions of base pairs, while GMOs involve the introduction of a foreign genetic material or transgene into the organism that may or may not be integrated into the genome (Callaway, 2018). Despite this fundamental difference, gene-edited organisms are often governed by the same set of rules and regulations as those for GMOs in many countries (El-Mounadi et al., 2020). For instance, the Court of Justice of the European Union (CJEU) had recently ruled that gene-edited crops are not exempted by laws and regulations governing GM crops (Callaway, 2018; Confédération paysanne and others v. Premier ministre and Ministre de l’Agriculture de l’Agroalimentaire et de la Forêt, 2018). This implies that the high hurdles that were put in place in developing GM crops also apply to CRISPR/Cas9-edited crops, which may drive funding and investment away from future research on CRISPR/Cas9 as a viable plant breeding technology. The EU’s unchanging definition of GMOs as “not naturally altered” further impacted the public perception toward CRISPR technology and genetic modification as a whole (Plan and Eede, 2010). The road to gain public confidence toward GMOs on their safety, efficacy, and benefits is already riddled with various aspects of social, economic, and legal challenges (Zimny et al., 2019). However, shifting the public perspective toward gene technology is the key to trigger much needed changes across the board.
In contrast to the EU, the US Department of Agriculture (USDA) ruled out regulation of genome-edited plants, provided its production does not involve plant pests (USDA, 2018). In addition to highlighting the safety and the lack of risks involved with genome-edited plants, this new ruling would promote further progress in the development of the technology (Hoffman, 2021). The first of the genome-edited crops allowed to bypass USDA regulations is a CRISPR/Cas9-edited white button mushroom resistant to browning (Waltz, 2016). The USDA has also been continuously funding research involving CRISPR-edited plants such as rice (O. sativa ) (Lee et al., 2019), pennycress (Thlaspi arvense L.) (Jarvis et al., 2021), and cocoa (Theobroma cacao) (Fister et al., 2018). Integrating modern technological approaches into regulations that were designed for older technology cannot possibly be the way forward. In contrast, law and regulations require modernization to keep up with the transformative power of innovation. Hence, rather than treating old GMO regulations as an umbrella that cannot continuously cover new and upcoming technologies such as CRISPR, regulations need to be amended as necessary.
However, despite periodic updates in GMO regulations and the development of novel guidelines, Malaysia is yet to approve the commercial growth of genome-edited crops (Singh et al., 2019). Similar to EU, Malaysia’s regulatory system classified genome-edited crops under GMOs; hence, any plant or crops would be difficult to gain approval by the system (El-Mounadi et al., 2020). Although Malaysia is relatively reserved in approving gene-edited crop propagation in the open field, it allowed more than 30 cases of import of transgenic products, albeit solely for the purpose of consumption or processing, in addition to approving confined field tests of transgenic plants such as rubber and papaya (Singh et al., 2019).
To be fair, crops produced by CRISPR/Cas9 gene editing and other gene editing methods utilized globally challenge the conventional perspectives and definition of gene modification and GMOs. Hence, there is no doubt that the regulatory bodies worldwide are still adapting to the rapid development of this technology. Therefore, despite legal hurdles, researchers, investors, and consumers alike should retain their interests in the development and research of more beneficial crops so that the supply would be able to cope with the rise in food demand.
Conclusions
CRISPR/Cas9 has received much attention in recent years as a revolutionary technology to genetically manipulate organisms to suit our demands. While initial research and development studied were focused on animal cell lines, the utilization of CRISPR/Cas9 gene editing has now been expanded to be inclusive of a diverse range of plant species, specifically beneficial and important crops. Through the enhancement of agricultural crops, agricultural and nutrition demands are expected to be met in an effort to improve the global quality of life. It has also been shown that the potential of CRISPR/Cas9 is not limited to the improvement of phenotypical traits, as this technology can also be used in live plant cell imaging to facilitate scientific research. There could be additional new methods to exploit CRISPR/Cas9 in the coming years, and this development should be anticipated in the fast-paced modernized era of scientific innovation. Therefore, scientific progress should not be discouraged or even impeded by issues concerning outdated regulation systems. This, coupled with low public acceptance and valuation of GMOs and CRISPR in general (Shew et al., 2018), indirectly influence the availability of funding toward further research. However, with patience and collaborative efforts from scientific community in sharing the knowledge and presenting advances in practical aspects of science, a shift in public perspective toward not just CRISPR/Cas9 but gene editing as a whole, would help to propel rapidly scientific progress in genome editing.