Introduction
Cirrhosis is a multifaceted clinical complication that affects many organs other than the liver (e.g., brain, kidney, heart, lung, and reproductive organs) [1-10]. On the other hand, cirrhosis-induced erythrocyte death and anemia are well-characterized complications of this disease that are approximately observed in 75% of cirrhotic patients [11-16]. The type of anemia, its etiology, and its prevalence are variable in cirrhotic patients (Table 1). Various forms of anemia, including normocytic (prevalence ≈40-54%), microcytic (≈20-28%), and macrocytic (≈44%), have been identified in cirrhosis (Table 1) [17]. The pathological changes in erythrocytes have been documented in various experimental models and human cases of cholestasis/cirrhosis [11-14, 16]. Several investigations have revealed that oxidative stress markers are significantly high in the erythrocytes isolated from cirrhotic patients [18]. Hence, oxidative stress and its consequences play a central role in cirrhosis-induced erythrocyte damage [11]. Significant changes in the membrane fluidity, along with increased oxidatively damaged proteins, have been found in the erythrocytes of patients with primary biliary cirrhosis [11]. Changes in erythrocyte thiols and protein sulfhydryl are also proposed to play a significant role in the susceptibility of erythrocytes of cirrhotic patients to damage and hemolysis [11]. Complications such as anemia might be improved upon liver transplantation as a gold-standard treatment for end-stage cirrhosis [16]. However, to our knowledge, this is the first report on the pharmacological options for preventing/alleviating erythrocyte damage in cholestatic/cirrhotic patients so far.
Table 1
Etiopathogenesis and prevalence of anemia in cirrhosis
Type of anemia | Etiology | Prevalence (%) | Reference(s) |
---|---|---|---|
Normocytic | Anemia of chronic disease (e.g., cirrhosis) | 40-54.1 | [158, 159] |
Microcytic | Portal hypertensive gastropathy | 20-80 | [160] |
Gastric antral vascular ectasia | 4 | [161] | |
Peptic ulcer | 35-53 | [158] | |
Hemolytic anemia in patients on interferon and ribavirin | 9-13 | [159] | |
Hemolytic anemia due to hypersplenism | 24 | [118] | |
Macrocytic anemia | Folic acid (vit. B9) deficiency | 44 | [158] |
Vit. B12 (cyanocobalamin) deficiency) | 31.8 in PBC 43 in NAFLD | [158] |
[i] This table is adapted from reference [17] (Creative Commons Attribution Non-Commercial; CC BY-NC 4.0). PBC – primary biliary cirrhosis, NAFLD – non-alcoholic liver disease
Taurine (TAU) is a cysteine-derived sulfur amino acid abundantly synthesized in the liver of several species, such as dogs and rats [19, 20]. Although TAU is the human body’s most abundant free amino acid, our liver’s capacity for TAU synthesis is negligible [21]. We obtain the majority of our body’s TAU from dietary resources [21]. TAU’s physiological and pharmacological properties have been widely investigated [20, 22-28]. For example, TAU has been found to be an osmoregulator, antioxidant, and regulator of mitochondrial function [20, 22, 26, 28-37].
Several studies have also mentioned the positive effects of TAU on erythrocytes [38-43]. In this regard, TAU significantly suppressed oxidative stress and its associated complications, such as lipid peroxidation and protein carbonylation in erythrocytes exposed to toxic insults [39-43]. TAU also significantly enhanced the level of antioxidant enzymes such as catalase, superoxide dismutase, glutathione-S-transferase, and glutathione peroxidase in erythrocytes [39, 40, 42, 43]. Moreover, TAU could enhance the total antioxidant capacity and reduced glutathione (GSH) levels in erythrocytes [39, 40]. Our research team used very high doses of TAU to mitigate cholestasis/cirrhosis-associated complications (e.g., cholemic nephropathy, skeletal muscle waste, cardiomyocytes injury, etc.) [44-50]. All these data reveal the potential protective properties of TAU in erythrocytes in various pathological conditions.
The current study aimed to evaluate the pathological changes in erythrocytes isolated from cholestatic/cirrhotic animals and assess the potential therapeutic role of TAU administration against this pathological condition. The data obtained from this study could help develop novel strategies for managing cirrhosis-associated clinical complications such as erythrocyte hemolysis and anemia.
Material and methods
Chemicals
Meta-phosphoric acid, 2-mercaptoethanol, 2,7-dichlorofluorescein diacetate (DCF-DA), 2,4,6-Tris(2-pyridyl)-s-triazine (TPTZ), ethylenediamine-tetraacetic acid (EDTA), GSH, ferric chloride hexahydrate (FeCl3.6H2O), and 5,5-dit hiobis-2-nitrobenzoic acid (DTNB) were obtained from Sigma-Aldrich (St. Louis, MO, USA). Sodium and potassium phosphates, saponin, trichloroacetic acid, sodium hydrogen phosphate dibasic, thiobarbituric acid, sodium chloride, sodium citrate, sodium hydrogen phosphate monobasic, n-butanol, methanol, and hydroxymethyl aminomethane hydrochloride (Tris-HCl) were obtained from Merck (Darmstadt, Germany). G6PD activity was determined using a commercial kit (Randox Laboratories, Antrim, United Kingdom). Commercially available Giemsa was purchased from ATR-MED (Tehran, Iran).
Animals
Mature male Sprague-Dawley (SD) rats (weighing 250 ±30 g) were obtained from the laboratory animals breeding center of Shiraz University of Medical Sciences, Shiraz, Iran. Animals were kept in a standard environment (temperature 25 ±1°C, 12 : 12 light : dark cycle, and ≈43 ±3% relative humidity). This investigation was conducted in compliance with the ARRIVE guidelines. Rats had free access to water and a standard laboratory animal diet (Behparvar, Tehran, Iran). The institutional ethics committee approved all experimental animal care and used procedures at Shiraz University of Medical Sciences, Shiraz, Iran (Registered ethical code: IR.SUMS.REC.1400.057).
Bile duct ligation surgery
Animals were randomly allotted to control (shamoperated) and bile duct ligated (BDL) groups. In the BDL group, rats were anesthetized using a mixture of 7 mg/kg of xylazine and 70 mg/kg of ketamine (i.p.). An incision (≈2 cm) was made through the linea alba, and the common bile duct was exposed and doubly ligated (silk suture; No. 04) [51-56]. The sham operation involved laparotomy without bile duct ligation [5, 57-62]. Animals were allowed to recover under infrared light in separate cages with free access to food and water [54, 63]. Rats were monitored at scheduled intervals to evaluate the pathogenic changes in blood parameters (3, 7, 14, 28, and 42 days after the BDL surgery). It was found that the maximum pathological changes in blood parameters of BDL rats were achieved on day = 42 after the BDL operation. Therefore, in another round of experiments, the following treatments were studied (7 rats/group): BDL + taurine (0.25% w : v in drinking water for 42 consecutive days); BDL + taurine (0.5% w : v in drinking water for 42 consecutive days); and BDL + taurine (1% w : v in drinking water for 42 consecutive days).
Erythrocytes’ isolation and plasma biochemical measurements
Blood samples (5 ml) were obtained from the abdominal aorta, transported to EDTA-coated tubes (Guangzhou, China), and centrifuged (3000 g, 15 min, 4°C). Aliquots (200 µl) of whole blood were also separated to determine G6-PD activity, blood parameters, and complete blood count (CBC). Then, blood samples were centrifuged (12,000 g, 15 min, 4°C), and the plasma was isolated by aspiration and used for biochemical analysis [64, 65]. Afterward, the erythrocytes were washed with phosphate-buffered saline (PBS) three times (3000 g, 10 min, 4°C). Finally, erythrocytes were suspended in PBS (5% v : v cell suspension) [43]. Hemoglobin concentration was measured in all suspensions for standardization of the obtained data. Commercial kits (Pars Azmun, Tehran, Iran) and a Mindray BS-200 autoanalyzer (Guangzhou, China) were employed to assess plasma γ-glutamyl transpeptidase (γ-GT), total bilirubin, alkaline phosphatase (ALP), alanine aminotransferase (ALT), and aspartate aminotransferase (AST) [64, 66]. Hematologic parameters were determined using Sysmex XS-800i (laser-based photometry) [67]. The G6-PD activity in rat erythrocytes was spectrophotometrically analyzed by a commercial kit (Pars-Payvand Saba, Tehran, Iran).
Light microscope morphological analysis of erythrocytes
Erythrocyte samples (100 µl) were suspended in 900 µl of PBS), and a smear was prepared. Then, samples were microscopically analyzed under a phase contrast microscope (Nikon Eclipse Ti-U inverted microscope; 100× magnification). Microcystic hypochromic erythrocytes and erythrocyte polychromasia were monitored.
Reactive oxygen species in the erythrocytes
The formation of reactive oxygen species (ROS) in erythrocytes was assessed using 2,7-dichlorofluorescein diacetate (DCF-DA) as a fluorescent probe [63, 68-75]. For this purpose, 500 µl of the 5% v : v (in PBS) of erythrocytes were suspended in 500 µl of 4°C Tris-HCl buffer (pH = 7.4, 40 mM). Then, 10 µl of DCF-DA (final concentration of 10 µM) was added and incubated in the dark (10 min, 37°C, shaking incubator) [65, 71, 76-83]. After incubation, the fluorescence intensity was measured at λexcit = 485 nm and λemiss = 525 nm using a fluorimeter (FLUOstar Omega, Germany) [84-91].
Erythrocytes’ glutathione content
Reduced glutathione concentration was determined by a method previously described based on the 5,5’-di- thiobis-2-nitrobenzoic acid method [43, 71, 92-94]. Briefly, 0.5 ml aliquots of RBC suspension in PBS were treated with 1 ml of ice-cooled distilled water, and 100 µl of trichloroacetic acid (20% w : v, 4°C). Samples were mixed well and centrifuged (16,000 g, 5 min, 4°C). Then, the supernatant was treated with 1 ml of Tris-HCl (pH = 8.9) and 100 µl of DTNB (20 mg/5 ml methanol). Finally, the absorbance was measured (λ = 412 nm, EPOCH) [43, 71, 95-97]. The GSH concentration was expressed in µmol/mg hemoglobin (Hgb).
Lipid peroxidation in isolated erythrocytes
Lipid peroxidation of erythrocyte membranes was assessed based on the thiobarbituric acid reactive substances (TBARS) assay method [71, 98-102]. For this purpose, aliquots (500 µl) of a 5% suspension of erythrocytes (in PBS) were treated with 250 µl of 20% trichloroacetic acid and 600 µl of 1% thiobarbituric acid. Samples were heated in a 100°C water bath for 15 minutes and then cooled (5 min at 0°C) [103-110]. Tubes were centrifuged (16,000 g, 5 min), and the absorbance of the supernatant (at λ = 532 nm) was measured [55, 71, 98, 111]. The concentration of TBARS was expressed in nmol/mg Hgb.
Results
Plasma biochemical measurements indicated an increase in liver injury biomarkers and a dramatic increase in plasma bilirubin and bile acids at different time intervals after BDL surgery (Fig. 1A). Liver tissue histopathological alterations also revealed significant collagen deposition and fibrosis in this organ (Fig. 1B). These data could confirm the appropriate induction of cholestasis/cirrhosis in the current BDL model. The maximum collagen deposition and liver fibrosis were achieved on day 42 after BDL induction (Fig. 1B).
Fig. 1
Plasma biochemical analysis (A) and liver tissue histopathological alterations (B; blue colored, green arrow) in bile duct ligated (BDL) rats. These data confirm the occurrence of cholestasis in the current model. Data are shown as mean ±SD (n = 7). Data sets with various alphabetical superscripts differ significantly (p < 0.05). Scale bars for histopathological images are = 100 µm
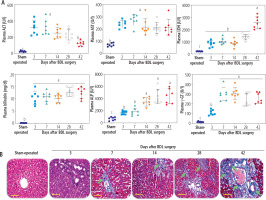
Blood analysis (CBC) of BDL rats revealed a significant decrease in erythrocyte count on day 42 after the BDL operation (Fig. 2). Other factors, such as Hgb and hematocrit (HTC), followed the same pattern and significantly decreased 42 days after BDL surgery (Fig. 2). Our model detected no significant changes in white blood cells (WBC) and platelet count at different intervals after BDL induction (Fig. 2). The effects of TAU on blood parameters were evaluated in BDL animals (Fig. 3). It was found that TAU (0.25%, 0.5%, and 1% w : v in drinking water for 42 consecutive days) significantly improved blood parameters in cirrhotic animals, including Hgb, HTC, and erythrocyte count. It should be mentioned that the effects of TAU on these parameters were not dose-dependent in the current model (Fig. 3).
Fig. 2
Complete blood count (CBC) and hematological alterations at different time points after bile duct ligated (BDL) surgery. A significant decrease in parameters associated with red blood cells (erythrocyte count, Hgb, and HCT) was detected in BDL rats 42 days after the BDL operation. No significant changes in WBC and platelets were detected in the current model. Data are presented as mean ±SD (n = 7). Data series with different alphabetical superscripts are significantly different (p < 0.05)
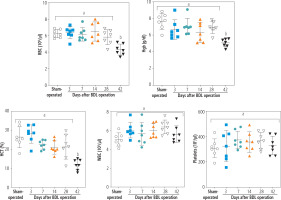
Fig. 3
Role of taurine (TAU) on complete blood count (CBC, RBC) of bile duct ligated (BDL) animals (42 days after BDL surgery). Data are represented as mean ±SD (n = 7). Data series with different alphabetical superscripts are significantly different (p < 0.05)
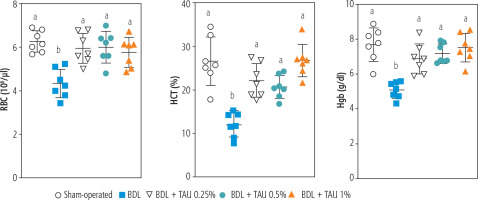
The activity of the enzyme G6PD in erythrocytes was another parameter assessed in the BDL animals. It was found that G6PD activity was significantly suppressed 42 days after the BDL operation. On the other hand, TAU (0.25%, 0.5%, and 1% w : v in drinking water for 42 consecutive days) significantly restored the G6PD activity in erythrocytes isolated from cirrhotic animals. No difference between various concentrations of TAU in improving G6PD activity was detected in the current study (Fig. 4).
Fig. 4
Monitoring glucose-6-deficiency (G6PD) at different intervals after bile duct ligation (BDL) surgery. A significant decrease in G6PD deficiency was detected 42 days after the BDL operation. It was found that taurine (TAU) significantly improved G6PD deficiency at both doses (0.25%, 0.5%, and 1% w : v). Data are shown as mean ±SD (n = 7). Data series with different alphabetical superscripts are significantly different (p < 0.05)
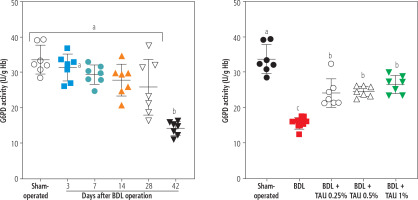
Morphological assessment of erythrocytes revealed the presence of hypochromic and polychromasia erythrocytes in the blood of BDL rats (42 days after BDL surgery; Fig. 5). No significant morphological changes in the blood of BDL rats were detected on days 3, 7, and 14 after the BDL operation (Fig. 5). It should be noted that TAU did not change erythrocyte morphological changes in the current model (data not shown).
Fig. 5
The light microscope analysis of erythrocytes in bile duct ligated (BDL) rats. A significant increase in microcytic hypochromic and polychromasia erythrocytes was detected in BDL rats. Taurine (TAU) had no significant effect on the RBS morphology in the current investigation. Data are shown as mean ±SD (n = 7). Data series with different alphabetical superscripts are significantly different (p < 0.05)
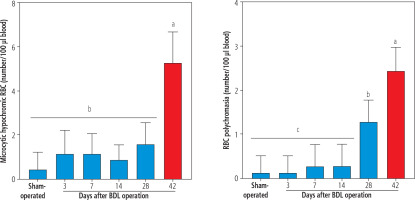
The assessment of biomarkers of oxidative stress at 28 and 42 days after BDL revealed significant ROS formation and lipid peroxidation in erythrocytes isolated from BDL rats. Moreover, the GSH level, CAT activity, and SOD activity of erythrocytes were significantly decreased in cirrhotic animals. It was found that TAU (0.25%, 0.5%, and 1%) significantly blunted markers of oxidative stress in erythrocytes of BDL rats. The effect of TAU on oxidative stress biomarkers was not dose-dependent in the current study (Fig. 6). As mentioned in various parts of the results, the effects of various doses of TAU (0.25%, 0.5%, and 1% w : v) on blood parameters assessed in the cirrhotic rats was not dose-dependent. Hence, the lower doses of this amino acid could be used for further investigations because of economic issues as well as preventing any potential adverse effects.
Fig. 6
Oxidative stress markers were assessed in erythrocytes on days 28 (A) and 42 after bile duct ligated (BDL) surgery (B). It was found that taurine (TAU; 0.5% and 1% w : v) significantly decreased biomarkers of oxidative stress in cirrhotic rats. In the current experiments, most oxidative stress markers were not significantly changed at 3, 7, and 14 days after cholestasis induction. Data are shown as mean ±SD (n = 7). Data series with different alphabetical superscripts are significantly different (p < 0.05)
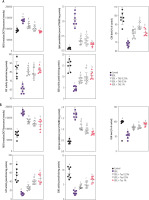
Discussion
The cirrhosis-induced hematological complication is a severe clinical problem [11-16]. On the other hand, oxidative stress and its associated complications could play a significant role in this situation [11]. Several case reports of cholestasis/cirrhosis-induced anemia have been published [112-114]. Hypochromic microcytic anemia seems to be humans’ most prevalent phenotype of cholestasis/cirrhosis-induced anemia [112]. In the current study, we detected microcytic hypochromic RBCs in the BDL rats 42 days after the BDL operation (Fig. 5). This finding is in line with other studies that indicate microcytic anemia as a prevalent type of morphological change of RBCs in cirrhosis [112]. On the other hand, detecting a significant number of polychromasia erythrocytes was another interesting finding that was detected 28 and 42 days after BDL induction (Fig. 5). Polychromasia occurs when RBCs are prematurely released from the bone marrow to the blood stream [115]. We did not find any relevant study on the etiology of polychromasia in the BDL animal model of cirrhosis. Nevertheless, it has been reported that polychromasia is frequently detected in patients with chronic hepatitis or hepatocellular carcinoma [115]. The mechanism(s) of releasing such erythrocytes in the current model is ambiguous and warrants further studies. Low hemoglobin levels (e.g., 6 g/dl) have also been reported in cholestatic patients with anemia [112]. As mentioned, oxidative stress and its associated complications play an essential role in erythrocyte damage in cirrhosis. Therefore, it is important to find safe and clinically applicable agents for preventing erythrocyte disruption. The data obtained from this study revealed that TAU, as a safe amino acid with exciting features such as membrane stabilization, antioxidant action, and osmoregulatory properties, significantly protected erythrocytes in cirrhotic rats.
Cirrhosis-induced erythrocyte damage and lysis are widely investigated. Several mechanisms have been proposed to be involved in this complication. In this regard, oxidative stress and its associated complications in erythrocytes have received much attention [116-118]. Erythrocytes continuously transport a high concentration of oxygen over their lifespan. Therefore, it is well known that these cells are susceptible to exogenous and endogenous reactive species and oxidative stress [119]. However, different effective antioxidant systems have been developed during the evolution of these cells to counteract oxidative stress in erythrocytes [120, 121]. This system is mainly involved in the presence of an enzyme known as glucose-6-phosphate dehydrogenase (G6PD) (Fig. 7) [122, 123]. This enzyme reduces oxidized NADP+ to its reduced form (NADPH). The enzyme glutathione reductase uses NADPH to convert oxidized glutathione (GSSG) to its reduced form (Fig. 7). GSH and its associated enzymes are vital for counteracting oxidative stress in erythrocytes [124, 125]. GSH is widely used in erythrocytes to detoxify reactive species directly or as a substrate for GSH-dependent antioxidant enzymes (Fig. 7) [124, 125]. Many experimental models also mentioned decreased erythrocyte antioxidant levels (CAT, SOD, glutathione peroxidase [GPx]) [121, 126]. It is well known that SOD is the most crucial antioxidant in erythrocytes [127]. Superoxide anion (O2•-) is the most dangerous reactive species that damages erythrocytes and causes deformability [127].
Fig. 7
Several options have been developed for managing anemia in cirrhosis in clinical settings. This figure is adapted from reference [17] (Creative Commons Attribution-NonCommercial; CC BY-NC 4.0); d – day, f – folic acid, b – bolus dose
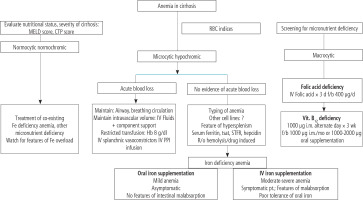
Another critical issue regarding erythrocytes is their membrane disintegrability [128, 129]. Several mechanisms have been proposed in experimental models and human cases of this disease. For example, Verkleij et al. found that the fusion of abnormal plasma lipoproteins could lead to abnormal and enlarged RBCs in cholestatic patients [129]. In another study, Okano et al. found that lecithin and fatty acid composition of erythrocyte membrane are changed in biliary obstruction [128]. All these events could destabilize the erythrocyte membrane, leading to its deformities and rupture (Fig. 7). An exciting feature of TAU is its effect on cell membranes. It is well known that TAU is a membrane stabilizer [130]. The membrane stabilizing action of TAU on erythrocytes could render their membrane more resistant to reactive species such as ROS and lipid peroxidation byproducts [131-133]. Gossai et al. also found that TAU significantly stabilized the erythrocyte membrane and enhanced cellular antioxidant capacity [134]. Excitingly, Gossai et al. also found that TAU effectively prevents Hgb and lactate dehydrogenase (LDH) release from erythrocytes [134]. They administered TAU (300 mg/kg) to diabetic rats. The formation of ROS, oxidized glutathione, and malondialdehyde was significantly decreased in the erythrocytes of TAU-treated mice [134]. Moreover, levels of catalase, glutathione peroxidase, and superoxide dismutase were considerably higher in the RBC of diabetic erythrocytes [134]. In another study by Gossai et al., the turnover of GSSG to GSH in erythrocytes reduced oxidative stress [134].
An important mechanism proposed for erythrocyte death and anemia in cholestasis/cirrhosis is proposed by Lang et al. [135]. They investigated the pathogenic effect of bilirubin on erythrocytes. In the current investigation, we found that the level of plasma bilirubin was very high in BDL rats (> 10 mg/dl) (Fig. 1). Lang et al. found that mechanistically bilirubin caused a severe influx of Ca2+ to erythrocytes, accumulation of sphingomyelinase activation, formation of ceramide, and translocation of phosphatidyl serine to the erythrocyte surface [136]. They concluded that all these events could lead to erythrocyte death and anemia in cholestasis/cirrhosis. Interestingly, the protective effects of TAU against Ca2+ effects on many cells is an essential feature of this amino acid [136]. Hence this characteristic of TAU could play an essential role in its protective effect on erythrocytes of cirrhotic animals in the current study.
As mentioned, RBCs are continuously exposed to exogenous and endogenous oxidants with different etiologies [137]. Oxidative stress could cause a decrease in the erythrocyte’s life span, hemolysis, and, finally, erythrocyte death. In this regard, a plethora of investigations have studied the effects of antioxidant molecules against this complication. Several studies have mentioned the protective properties of antioxidant molecules such as β-carotene, polyphenols (e.g., resveratrol and quercetin), methionine, α-lipoic acid, N-acetylcysteine, melatonin, dithiothreitol (DTT), and homocysteine on erythrocytes damage with different etiologies [138-144]. Based on these data, using these molecules could significantly protect erythrocytes in various pathological conditions. Several options have been developed for managing anemia in cirrhosis in clinical settings (Fig. 7) [17]. Based on the study by Singh et al. [17], complementary treatment strategies could play a role in alleviating cirrhosis-induced anemia. First, removing the background diseases such as hepatitis or alcoholism might be very helpful [17]. Second, dietary advice (e.g., administration of elemental iron and other micronutrients) could help alleviate cirrhosis-induced anemia (Fig. 7). On the other hand, several studies have revealed the exciting effects of TAU on erythrocytes [38-43]. TAU is not considered a classic radical scavenger or a regulator of cellular antioxidant defense mechanisms [145-147]. Actually, TAU acts as a regulator of cellular ROS generation [145, 146, 148]. There is a plethora of evidence indicating the role of TAU in regulating mitochondria-mediated ROS formation [145, 146]. However, this mechanism is irrelevant to cells devoid of mitochondria (e.g., erythrocytes). Hence, another mechanism should be involved in the protective properties of TAU in erythrocytes. Interestingly, it has been proposed that TAU could have a regulatory effect on mitochondrial defense mechanisms or even the expression of the action of oxidants on mitochondrial-encoded proteins [149]. Then, the direct regulatory properties of TAU and its effects on cellular antioxidant mechanisms could play a pivotal role in its protective properties in biological systems.
Interestingly, the effect of TAU on erythrocytes’ G6PD enzyme activity has also been reported in previous studies [150, 151]. It was found that TAU significantly prevents suppression of the G6PD enzyme activity in erythrocytes exposed to toxic levels of cadmium, hydrogen peroxide, phenylhydrazine, and lead. In some studies it has been found that TAU could significantly increase the ratio of GSH/GSSG, which could directly increase the direct radical scavenging activity of GSH or indirectly enhance the activity of GSH-dependent enzyme systems (Fig. 7). Moreover, isolated erythrocytes have been challenged with H2O2 as a potent antioxidant [150, 151].The researchers also detected that TAU significantly prevented erythrocyte ATP breakdown, promoted GSH levels, and enhanced the pentode phosphate pathway [150, 151]. In the current investigation, we found that the oxidative stress markers were significantly elevated in cirrhotic rats. Another exciting finding of our study was the positive effects of TAU on the G6PD enzyme activity in erythrocytes of cirrhotic animals (Figs. 4 and 8). The higher activity of G6PD boosts erythrocytes’ antioxidant system and prevents cell death (Figs. 7 and 8). We also found that TAU significantly improved the antioxidant activity of erythrocytes isolated from cirrhotic animals (Fig. 6).
Fig. 8
Oxidative stress and its associated complications play a critical role in the pathogenesis of cirrhosis-induced erythrocyte damage, lysis, and anemia. The accumulation of potentially cytotoxic molecules, such as hydrophobic bile acids and supraphysiological concentrations of bilirubin, could be involved in the pathogenesis of these adverse effects
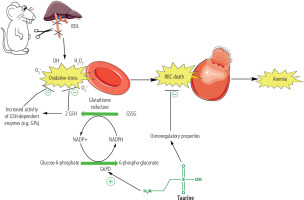
Another critical issue in erythrocyte storage and, finally, transfusion to recipients is the oxidation of the erythrocyte membrane. The oxidation of fatty acids ultimately compromises the stability of the erythrocytes’ membrane and eventually impairs their activity [152-154]. Interestingly, TAU is an excellent membrane stabilizer [130, 149, 155-157]. It has repeatedly been mentioned that TAU protects biomembrane lipids from oxidation and consequently prevents cell damage [130, 149, 155-157]. Huxtable et al. mentioned that inhibiting lipid peroxidation, stabilization of biological membranes, TAU antioxidant properties, and maintaining intracellular ions’ homeostasis play a crucial role in preserving cellular integrity and preventing their damage [23]. In the current study, we found that TAU treatment significantly mitigated hemolysis and lipid peroxidation in erythrocytes of cirrhotic animals (Fig. 6). Hence, this exciting feature of TAU could effectively prevent erythrocyte damage and hemolysis and play a crucial role in its protective properties in cirrhosis.
Treatments for cholestasis/cirrhosis-induced erythrocyte damage and anemia are symptomatic, and there are no specific pharmacological interventions to prevent or treat this complication. Hence, the data from this study might pave the way for finding novel and clinically applicable therapeutic options for preventing cholestasis/cirrhosis-induced erythrocyte damage and anemia (Fig. 8). Further studies are warranted to explain the precise mechanisms of TAU’s protective properties against erythrocyte damage and its application in clinical settings in cirrhotic patients, blood transfusion, and/or blood diseases with susceptible erythrocytes.