INTRODUCTION
Although many hypoxic training methods exist, the majority of endurance athletes still utilize altitude training with prolonged exposure to moderate hypoxia (living high, training high) [1]. However, more and more elite athletes who need to repeat high intense efforts during competition include intermittent hypoxic training methods in their training, and, more specifically, high-intensity interval training in hypoxia (HIIT).
Such intermittent hypoxic training has been shown to augment oxidative stress compared to similar training done in normoxia [2]. Indeed, aggravation of oxidative damage through unbalanced DNA strand breakage [3], increase in lipid peroxidation [4] and protein oxidation have been reported under exercise in hypoxic conditions. Although all mechanisms underlying this unnecessary oxidative stress are not entirely clear, they lead to reduced redox potential within the mitochondria as well as increased catecholamine production and activation of the xanthine oxidase pathway (see [2] for more details). This could also be the consequence, not only of the increased activity of ROS generated, but also of the decreased activity of antioxidant systems [5].
Antioxidant supplementation can have beneficial effects in attenuating and/or preventing oxidative damage associated with exercise in hypoxia. Nitric oxide (NO), as an important antioxidant agent that suppresses the formation of free radicals [9], through the NO uncoupling pathway, would be a worthy intervention. However, its bio-availability can be enhanced through an alternative pathway involving sequential reduction of nitrate (NO3−) to nitrite (NO2−) and further to NO. The latter is independent of oxygen [6], and therefore augmented in hypoxic conditions. Reducing ROS formation is expected to be beneficial, especially mediated by the oxygen independent NO3− – NO2− – NO pathway. Therefore, increasing NO bio-availability of this pathway may provide potential ergogenic effects for exercise performed in hypoxia, as the availability of NO has been suggested to influence human acclimatisation to altitude [7]. Not-withstanding this hypothesis, there is little evidence on the influence of NO3− supplementation on oxidative stress induced by hypoxic high-intensity exercise. Ashmore et al. [8] studied rats exposed to normobaric hypoxia, reporting that dietary NO3− supplementation reduced the levels of oxidative stress markers at rest, suggesting that this strategy may be of benefit to individuals exposed to altitude. Carriker et al. [9] investigated changes in oxidative stress and arterial oxygen saturation (SaO2) during exercise in hypobaric hypoxia following acute NO3− supplementation in well-trained males and concluded that acute NO3− supplementation yielded no beneficial changes in oxidative stress. However, to our knowledge, the influence of NO3− supplementation on oxidative stress during a longterm period of high-intensity exercise in hypoxia remains unknown.
Whereas the acute combination of both hypoxia and exercise (of low, moderate and high intensities) clearly augments oxidative stress [2, 10] long-term responses to combined stimuli remain debated. In particular, high-intensity training (work performed above the lactate threshold interspersed by periods of low-intensity exercise or complete rest) has been shown to significantly augment oxidative stress mostly via reduced antioxidant capacity [11, 12]. In contrast with these findings, moderate intensity exercise does not seem to modify antioxidant status or significantly alter redox balance [13]. It must be noted that during these interventions the “living high, training low” model was implemented, and therefore exercise sessions were performed in normoxia. Collectively, this suggests that long-term high-intensity training under hypoxic conditions (training high) may regulate even more the systemic redox balance in humans. Therefore, increasing NO bioavailability, via the NO3− – NO2− – NO pathway, may provide a key complement in reducing oxidative stress induced by exercise (especially at high intensity) in hypoxia.
The aim of the present study was therefore to analyse the effects of dietary NO3− supplementation combined with prolonged high-intensity training performed under normobaric hypoxic conditions on antioxidant/pro-oxidant balance. It was hypothesized that enhancing NO production via the NO3− – NO2− – NO pathway, by dietary NO3− supplementation, would mitigate oxidative stress under hypoxic conditions.
MATERIALS AND METHODS
Subjects
Thirty trained, developmental age male subjects (mean ± SD: 54.4 ± 8.2 ml · kg · −1min · −1, 36.2 ± 6.3 yrs., 71.5 ± 8.1 kg and 174.8 ± 6.8 cm for relative maximal oxygen uptake:
Intervention
This randomized, single-blind, placebo-controlled, independent group study was conducted in a normobaric hypoxic facility (Porto’s Exercise Medical Center, Portugal: b-Cat). Participants performed (cycle ergometer) 12 high-intensity interval training (HIIT) sessions during a 4-week period (3 sessions/week), while randomly assigned to one of three experimental groups: (i) HNO: high-intensity exercise training sessions in normobaric hypoxia (FiO2 = ~13%, ~3000 m) with NO3− supplement; ii) HPL: high-intensity exercise training sessions in normobaric hypoxia (FiO2 = ~13%, ~3000 m) with placebo supplement, and iii) CON: high-intensity exercise training sessions in normoxia (FiO2 = 20.9%) with placebo supplement.
Subjects were instructed to maintain their habitual physical activity level and normal diet but were asked to abstain from the use of any chewing gum and antibacterial mouthwashes products [15]. In the week before (baseline) and after the 4-week period (post-intervention; between the second and third day after the last training session), venous blood samples were collected (in the late afternoon/early evening period) from the median cubital vein and participants performed an exercise transition from rest to severe intensity until exhaustion (Tlim) on a cycle ergometer (Lode Excalibur Sport, Groningen, The Netherlands).
Each week, participants performed two sessions of short aerobic intervals (HIT: 2 sets of 6 × 1 min at 90%Δ with 1 min active recovery between repetitions and 3 min between sets) and one session of repeated sprint training (RST: 4 sets of 6 × 10 s “all-out”, with 20 s active recovery and 3 min between sets) on a cycle ergometer (Lode Excalibur Sport, Groningen, The Netherlands). All training intensities used were relative to specific p
Measurements
Time sustained was determined through the Tlim test and performed at 80%Δ, as previously reported [17]. The test ended when the cadence could no longer be maintained within 10 rpm of the preferred cadence for > 5 s. Before the test, a standard 5 min warm-up exercise (50% of p
After venous blood samples were withdrawn, plasma was immediately separated by centrifugation (10 min at 3000 g; 4°C). Then samples were stowed in aliquots and immediately stored at -80ºC until analysed for: i) subsequent oxidative stress markers (advanced oxidation protein products: AOPP, malondialdehydes: MDA, nitrotyrosine, ferric-reducing antioxidant power: FRAP, and uric acid: UA), ii) antioxidant enzymes (superoxide dismutase: SOD, catalase, glutathione peroxidase: GPX, and myeloperoxidase: MPO) and, iii) nitric oxide metabolites (NO3−, NO2− and NOx). All assays on plasma sample were conducted by spectrophotometry.
Data Collection Procedure
Raw SmO2 and THb data were treated using a smooth spline filter to reduce the noise created by movement and data presented every 2 s. Baseline SmO2 (SmO2base) and baseline THb were computed as a 30-s average while subjects performed 3 min of unloaded baseline pedalling (8 W) at their preferred cadence before the beginning of each test. Minimum SmO2 (SmO2min) was the lowest 6-s average obtained during each test. Maximum SmO2 (SmO2max) and maximum THb were the highest 6-s average obtained during each test with the recovery phase included. Average SmO2 from 30 to 120 s after the end of each test was used to assess recovery of SmO2 (SmO2recovery). For each test, baseline SmO2base and SmO2min are expressed as % of SmO2max (relative-SmO2base and relative-SmO2min, respectively). Change in SmO2 (DSmO2) and change in THb DTHb were calculated as the difference between relative SmO2min and relative SmO2base and the difference between maximal and baseline THb [18].
Blood Samples
Catalase activity in the plasma was determined using H2O2 as a substrate and formaldehyde as a standard. Catalase activity was determined by the formation rate of formaldehyde induced by the reaction of methanol and H2O2 using catalase as the enzyme (intra-assay coefficient of variation: CV = 3.1%). GPX activity was determined as the rate of oxidation of NADPH to NADP+ after addition of glutathione reductase (GR), reduced glutathione (GSH) and NADPH, using H2O2 as a substrate (intra-assay CV = 4.6%). SOD activity was determined by the degree of inhibition of the reaction between superoxide radicals, produced by the hypoxanthine—xanthine oxidase system, and nitroblue tetrazolium (intra-assay CV = 5.6%). MPO activity was measured in plasma by determination of the kinetic absorbance at 653 nm after addition of H2O2 and 3,3’,5,5’-tetra-methylbenzidine (TMB: intra-assay CV = 5.1%). FRAP concentration was calculated using an aqueous solution of known Fe2+ concentration (FeSO4, 7H2O2) as standard at a wavelength of 593 nm (intra-assay CV = 2.9%). The concentration of plasma UA was determined using a commercially available kit. The principle is: uricase acts on uric acid to produce allantoin, CO2 and H2O2. The absorbance is proportional to the uric acid quantity in the sample (intra-assay CV = 0.9%). AOPP assay was calibrated with a chloramine-T solution that absorbs at 340 nm in the presence of potassium iodide. AOPP concentrations were expressed as μmolL−1 of chloramine-T equivalents (intra-assay CV = 5.4%). MDA concentration was determined by extracting the pink chromogen with n-butanol and measuring its absorbance at 532 nm by spectrophotometry using 1,1,3,3-tetrae-thoxypropan as standard (intra-assay CV = 2.2%). The metabolites of NO, NO2− and NO3− were measured using the reagent of Griess, a mixture of sulfanilamide, naphthalene ethylene diamine dihydro-chloride and phosphoric acid. This reagent binds nitrite to form a dye which absorbs at 550 nm. In a second measurement, the NO3− reductase was added to the plasma sample in order to convert NO3− into nitrite to measure the total amount of nitrites and nitrates (NOx) (intra-assay CV = 3.9, 5.2 and 4.8% for NO2−, NO3− and NOx, respectively). The plasmatic nitrotyrosine concentration was measured by the ELISA method (enzyme-linked immunosorbent assay: intra-assay CV = 6.8%).
Statistics
It was considered 10 participants per group for a type I error of 5%, a power of 80%, with statistical significance, and an average population effect size to be detected with probability of 0.5 (G*Power software version 3.1.9.2), considering the time-to-task failure variable at the severe intensity exercise domain. The Shapiro-Wilk test was used to confirm data normality and homogeneity. Data are presented as mean ± SD. Repeated measures analysis of variance (ANOVA) with two factors (group × time) was used to test main and interaction effects for the studied variables. A contrast analysis was used for post-hoc comparisons when an interaction effect was observed (Bon-ferroni test). Magnitudes of standardized effects (partial eta square – r2) were determined as follows: small, 0.2–0.5; moderate, 0.5–0.8, and large, > 0.8. All statistical procedures were conducted with SPSS 24.0 and the significance level was set at 5%.
RESULTS
NOx increased (+22%) between pre- and post-intervention periods, though not significantly (time effect: p = 0.06). Also, plasmatic nitrates (+21%) and nitrites (+33%) increased non-significantly (time effect: p = 0.07 and p = 0.09 for nitrates and nitrites, respectively). Only nitrotyrosine significantly decreased (time effect: p = 0.04) from pre- to post-intervention, regardless of the group (-22% in the HNO group, -41% in the HPL group and -45% in the control group) (Figure 1).
FIG. 1
Plasma concentration (mean + SD) of nitrate reductase (NOx: panel A), nitrates (panel B), nitrites (panel C) and nitrotyrosine (panel D) in pre-intervention (1 week before the beginning of training and supplemen-tation) and in post-intervention (1 week after the end of training but following supplementation) for high-intensity exercise training sessions performed in hypoxia with NO3-(HNO: black), in hypoxia with placebo (HPL: light grey) and in normoxia with placebo (CON: dark grey).
Significant differences: * Compared to Pre (p<0.05)
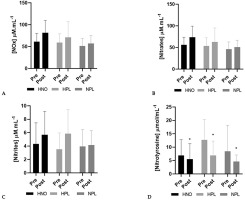
There was a time effect for GPX (+12%, p = 0.025), increasing but only in CON (p = 0.017, 20%) and not in the HNO and HPL groups. In addition, at post-intervention, GPX activity in plasma was lower for HPL compared to HNO (p = 0.04) and CON (p = 0.01) groups (Figure 2A). In contrast, SOD, catalase and FRAP concentration were not modified between pre- and post-intervention for the 3 groups (Table 1).
TABLE 1
Mean ± SD superoxide dismutase activity (SOD), catalase concentration and ferric reducing ability power (FRAP), advanced oxidation protein product (AOPP), myeloperoxidase (MPO) and uric acid activity at pre-intervention (1 week before the beginning of training and supplementation) and post-intervention (1 week after the end of training but following supplementation) for high-intensity exercise training sessions performed in hypoxia with NO3− (HNO), in hypoxia with placebo (HPL) and in normoxia with placebo (CON)
FIG. 2
Plasma activity (mean + SD) of glutathione peroxidase enzyme (GPX: panel A) and plasma concentration of malondialdehyde (MDA: panel B) in pre-intervention (1 week before the beginning of training and supplementation) and in post-intervention (1 week after the end of training but following supplementation) for high-intensity exercise training sessions performed in hypoxia with NO3- (HNO: black), in hypoxia with placebo (HPL: light grey) and in normoxia with placebo (CON: dark grey).
Significant differences: * Compared to Pre (p<0.05); # HNO post > HPL post (p<0.05); $ CON post > HPL post (p<0.05)
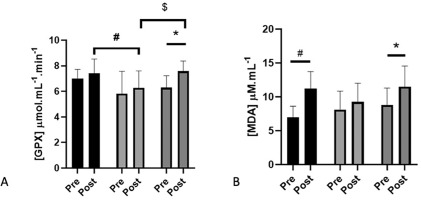
There was a time effect for MDA (+34%, p = 0.0003). MDA increased in HNO (+60%; p = 0.001) and in CON (+30%; p = 0.023) but not in the HPL group (Figure 2B). Conversely, AOPP, uric acid, and myeloperoxidase activity were not modified by the protocol whatever the group (Table 1).
SmO2recovery decreased (20%) from pre- to post-training (time effect: p = 0.01). However, the decrease was significant only in CON (58%; p = 0.0003) but not in HNO or HPL. In addition, at post-in-tervention, SmO2recovery was higher in HNO (p = 0.001) and HPL (p = 0.0006) than in the CON group (group effect: p = 0.02; interaction effect: p = 0.01) (Table 2). At post-intervention, SmO2base (25%; p = 0.007) and relative SmO2base (22%; p = 0.002) were lower in HPL compared to the CON group. This latter parameter was also lower in HPL (8%; p = 0.03) compared to the HNO group. SmO2min (61%; p = 0.003) and relative SmO2min (60%; p = 0.04) were lower in HNO compared to the CON group. In addition, DSmO2 was also lower in HNO compared to the HPL group (15%; p = 0.04) (Table 2).
TABLE 2
Mean ± SD values for oxygen saturation parameters obtained during the Tlim at pre- (1 week before the beginning of training and supplementation) and post-intervention (1 week after the end of training but following supplementation) for high-intensity exercise training sessions performed in hypoxia with NO3− (HNO), in hypoxia with placebo (HPL) and in normoxia with placebo (CON)
HNO | HPL | CON | ||||
---|---|---|---|---|---|---|
Pre | Post | Pre | Post | Pre | Post | |
SmO2base (%) | 71.44 ± 9.81 | 69.00 ± 11.36 | 62.00 ± 7.30 | 64.30 ± 8.77## | 71.70 ± 7.10 | 75.44 ± 6.31 |
SmO2min (%) | 5.44 ± 5.05 | 4.67 ± 3.35## | 5.14 ± 8.32 | 8.10 ± 9.72 | 8.20 ± 8.36 | 12.78 ± 9.63 |
SmO2max (%) | 80.78 ± 5.36 | 77.33 ± 8.20 | 78.71 ± 4.03 | 78.50 ± 5.50 | 82.60 ± 3.03 | 82.00 ± 6.61 |
SmO2recovery (%) | 72.82 ± 8.43 | 68.49 ± 13.78## | 69.72 ± 7.50 | 69.68 ± 8.76## | 71.83 ± 7.17 | 42.19 ± 34.09* |
Relative-SmO2base (% of SmO2max) | 88.17 ± 7.75 | 88.80 ± 6.96# $ | 78.69 ± 7.52 | 81.67 ± 6.49## | 86.73 ± 6.90 | 92.33 ± 8.14 |
Relative-SmO2min (% of SmO2max) | 6.58 ± 5.86 | 6.08 ± 4.24# | 6.63 ± 10.82 | 10.17 ± 12.28 | 9.95 ± 9.92 | 15.16 ± 11.25 |
DSmO2 (%) | -81.59 ± 8.37 | -82.71 ± 8.22$ | -72.06 ± 16.37 | -71.50 ± 12.49 | -76.78 ± 10.03 | -77.17 ± 14.41 |
[DTHb] (AU) | 0.12 ± 0.11 | -7.86 ± 23.74 | 0.13 ± 0.11 | 0.11 ± 0.12 | 0.22 ± 0.16 | 0.08 ± 0.20 |
DISCUSSION
The aim of this study was to analyse the effects of a prolonged combination of dietary NO3− supplementation with HIIT performed in normobaric hypoxia on antioxidant/pro-oxidant balance in male endurance subjects. It was hypothesized that dietary NO3− supplementation would mitigate the detrimental effect of hypoxia on oxidative stress, mainly by enhancing NO production via the NO3− – NO2− – NO pathway. Our main results showed that hypoxia inhibits the increase in GPX activity as only the CON group showed differences between pre-and post-intervention periods. However, our initial hypothesis that dietary NO3− supplementation would mitigate the detrimental effect of hypoxia on oxidative stress was confirmed. It appears that NO3− supplementation (in the HNO group) could compensate for the inhibitory effect of hypoxia (observed in HPL group), although hypoxia limited the increase in MDA in response to HIIT.
Our results confirmed that a normoxic HIIT increased GPX activity in plasma as already observed in untrained subjects [20], where-as in our study, the subjects were endurance trained. Hypoxia (with and without NO3− supplementation) attenuated this increase in GPX following 4 weeks of HIIT exposure. One may hypothesize that the total metabolic stimulus (exercise demands) of HIIT sessions performed in hypoxia was lower and induced lower mitochondrial ROS production [21, 22]. However, the total energy produced and the distance achieved in HIIT sessions (averaged over the 12 training sessions) were not different between the HNO, HPL and CON groups, suggesting that the relative exercise intensity performed was similar.
Our results of GPX are also contradictory to those showing that acute hypoxia increases oxidative stress and decreases the activity of antioxidant enzymes [23]. Only one study has measured the activity of antioxidant enzymes following a 3-week training program of HIIT in hypoxia [24]. These authors observed an increase in GPX activity from pre- to post-intervention. However, several differences may explain the discrepancy between this study and ours: the athletes were professional, with
Although several previous studies have reported that endurance training in normoxia [20, 25] or in hypoxia [26, 27] induces an improvement in the SOD and catalase activities, we did not observe any significant change for these two antioxidant enzymes. Nevertheless, it is important to emphasize that the work of Miyazaki et al. [20] was conducted with an untrained population, whereas the participants in the present study were endurance trained. Therefore, one may speculate that the activities of these antioxidant enzymes at the beginning of the intervention were already sufficiently high in our subjects compared to untrained subjects, thus contributing to limit their increase [2]. Antioxidant enzymes are higher for endurance athletes vs. sedentary or non-endurance/sprint athletes. In support of this, our results corroborate those of Robertson et al. [25], who did not report a significant improvement in SOD and catalase following HIIT in both normoxia and hypoxia in highly endurance-trained cyclists.
Hypoxia blunted the MDA increase induced by the training intervention (i.e., increase in CON group vs no change in HPL group). This could be a result of lower ROS production in mitochondria in hypoxia during the HIIT sessions. Unlike GPX, it appears that NO3− supplementation blunted the inhibitory effect of hypoxia on MDA increase after 4 weeks of HIIT training.
The first hypothesis would be that NO3− supplementation could have induced a higher intensity (i.e., power output) performed during HIIT sessions in hypoxia, as proposed by Cocksedge et al. [28]. This would therefore increase the production of ROS mainly from the upregulation of NADPH oxidase 2 and activation of the phospholipase A2 pathway. However, since there were no differences in energy (419.91 ± 52.76 vs. 442.04 ± 66.84 kJ) or distances covered (27999 ± 3576 vs. 29540 ± 4530 m) during the 12 training sessions between HNO and HPL, respectively, this mechanism should not be considered. It is also unlikely that the lipid content and the amount of plasma lipid peroxidation substrate were increased by NO3− supplementation. Indeed, such supplementation seems rather to lower plasma triglycerides and cholesterol [29]. Another hypothesis to explain the MDA increase in plasma might be related to the effects of NO3− on oxygen availability in muscles. In the HNO group, post-training SmO2min was lower than in the other groups (Table 2), suggesting that NO3− intake may induce greater vasodilation and therefore a greater oxygen delivery to active muscles in hypoxia [28, 30, 31]. This later mechanism during hypoxic exercise under NO3− supplementation would therefore induce greater ROS mitochondrial production and lead to an increase in the production of MDA.
The small effect of NO3− supplementation on the oxidative stress and antioxidant markers could be explained by the very modest increase in nitrates in the circulation. It should also be acknowledged that the blood samples were collected a few days after the last supplementation. It was reported previously that there was no additional improvement in exercise tolerance after ingesting beetroot juice containing 16.8 compared with 8.4 mmol NO3− over 24 h [32]. In this context, the nitrates dose in our study may therefore be too low (8.4 mmol before each session) to stimulate NO metabolism. The necessary dose of ingested nitrates to significantly increase plasma nitrate concentration, especially chronically, should be higher in endurance-trained athletes (0.07 mmol NO3−/kg body weight per day) [33]. This limited increase in plasma nitrate concentrations could be explained by the characteristics of our trained subjects, who usually present greater endothelial NOS (nitric oxide synthase) activity and therefore high endogenous NO production [34]. In addition, trained subjects have higher plasma nitrite concentrations than sedentary or active subjects [35], and also the response to a standard dose of nitrates may be lowered [36]. Finally, recent evidence showed that nitrate supplementation preferentially modified contractile function in type II fibres (vs. type I), which are in lower proportion in endurance athletes, likely explaining the limited physiological response to nitrate supplementation [37].
Regardless of the condition (hypoxia and/or NO3− supplementation), the 4 weeks of HIIT training had only minor effects on plasma nitrite levels. Our results confirm those obtained by Dreißigacker et al. [38] showing that high-intensity exercise in normoxia did not induce a significant increase in nitrites in trained cyclists. These authors suggested that nitrite was probably more reduced in NO in erythrocytes during high-intensity than during low-intensity exercises.
Through its ability to produce peroxynitrite by reacting with the superoxide anion, NO could also cause nitrosative stress on biomolecules and increase its end products, such as nitrotyrosine [39]. Nevertheless, we did not observe any significant change in plasmatic nitrotyrosine, either in the NPL and HPL groups, or in the HNO group. It has been observed in highly trained endurance athletes that NO3− supplementation containing 8 and 16 mmol of nitrates did not induce a significant increase in plasma peroxynitrite following an incremental and maximal effort on a treadmill [40]. On the other hand, when nitrate supplementation contained 24 mmol of nitrates, these authors observed a significant increase in plasma peroxynitrite. Therefore, the relatively low dose of nitrate (8.4 mmol) ingested before each training session by the participants of the HNO group may partly explain the lack of a significant effect of nitrate supplementation on plasma nitrotyrosine.
We did not detect any significant change in plasma AOPP and uric acid. To our knowledge, no study has measured the effects of such hypoxic training intervention (i.e., living low, training high) on plasma AOPP. In the context of the present study, one may hypothesize that the ROS generated during the training sessions was not sufficient to induce significant protein oxidation, regarding the enzymatic antioxidant capacities of the endurance-trained participants [2]. Finally, in agreement with our results, two recent studies have shown, in endurance-trained athletes, that HIIT training carried out in normoxia or hypoxia did not induce a significant modification of plasma uric acid [24, 40].
One may question the relevance of using time-to-exhaustion exercise since performance time is known as less reliable than for timetrial exercise. First, as reported by Hopkins et al. [41], the constant-power test is not better or worse than a constant-work or constant-duration test. When converted to mean power, constantload tests are more reliable than the other tests. Secondly, by definition, time-to-exhaustion exercise does not require the intensity to be paced. Consequently, this minimizes the potential changes in lactate production and in the oxidative-glycolytic balance of the exercise (known [42] to be one of the most important determinants of the physiological responses, including muscle de-reoxygenation) during high-intensity exercises in hypoxia.
In the present study we adopted an independent group design (not a crossover one), and although we ensured that all groups were blinded for both the intervention (normoxia vs. hypoxia conditions) and the supplementation (NO3− vs. placebo) conditions, the fact that some of the participants may have identified the group to which they were allocated may have slightly influenced the results obtained. In addition, it cannot be excluded that a higher daily dose of NO3− may have a more pronounced effect. Moreover, since heart rate, lactate or rate of perceived exertion responses are either directly influenced by hypoxia or are irrelevant for RST, the quantification of internal training loads is difficult in the present study. Also, it is important to bear in mind that although the NIRS device used in the present study is a valid and reliable one, there is still a need for further development of this equipment at higher intensities.
CONCLUSIONS
The present study focused on the effects of high-intensity normobaric hypoxic training associated with NO3− supplementation on oxidative stress, antioxidant defence and NO metabolism in endurance subjects. Normobaric hypoxic exposure during HIT and RST sessions blunted the increase in GPX and MDA at the end of the training period. In addition, since the NO3− supplementation used in the study had only a modest effect on plasma nitrate and nitrite contents, its effects only very slightly mitigate the detrimental effects of high-intensity training under normobaric hypoxic conditions on oxidative stress and antioxidant markers. Future studies should test a higher dose of NO3− to conclude whether NO3− supplementation can provide beneficial effects on the oxidative stress-NO metabolism axis in response to high intensity normobaric hypoxic training. Considering the latter, NO3− supplementation cannot be currently recommended to mitigate the detrimental effects of high-intensity training under hypoxic conditions on oxidative stress and antioxidant markers.