Introduction
The coronary artery bypass graft (CABG) surgery is a common revascularization procedure to rescue viable myocardium from impaired blood supply [1]. Ischaemia and reperfusion (I/R) injury of autologous graft veins during graft harvest and implantation is the most important factor increasing the risk of graft failure and the need for revascularization [2]. Vascular I/R injury can lead to endothelial dysfunction, the formation of toxic reactive oxygen species, the release of pro-inflammatory cytokines, and cell death [3–5].
The in vitro rat vascular I/R injury model is frequently used in the literature to evaluate the effects of pharmacological agents effective against this injury [6–10]. Although this model is generally considered valid, there is no consensus on whether hypoxia, rather than complete ischaemia, is sufficient to represent endothelial and vascular dysfunction induced by I/R injury of autologous graft veins [10–12]. Furthermore, it is also unclear to what extent the addition of reperfusion to hypoxia/ischaemia plays a role in vascular dysfunction. A closer look at the in vitro vascular I/R model will guide future studies that will investigate the effects of potential pharmacologic agents and preservation solutions on vascular I/R injury and explore the cellular and molecular mechanisms of I/R injury using this model.
Aim
In this study, we aimed to compare the effects of hypoxia and ischaemia, with or without reperfusion, on the vascular functions of isolated thoracic aortic rings of rats. We conducted our study on aged rats to represent aging-induced vascular damage in the elderly human population, where vascular I/R injury is more aggravated [13].
Material and methods
Animals
This experimental study was approved by Başkent University Ethical Committee for Experimental Research on Animals (project no. DA22/33, date 12/12/2022) and carried out in accordance with the 8th Edition of the Guide for the Care and Use of Laboratory Animals (National Research Council. Guide for the Care and Use of Laboratory Animals, 8th ed. Washington, DC: The National Academies Press, 2011).
Twelve male Sprague-Dawley rats (350–500 g, 18–24 months old) were housed under a 12-12 h light/dark cycle in a room with a controlled temperature (22 ±2°C). Animals accessed food and water ad libitum and were acclimated for at least one week before the start of the experiments.
Surgical procedure and preparation of aortic rings
The midline thoracic incision was performed under general anaesthesia (100 mg/kg ketamine i.p., 10 mg/kg xylazine i.p.), and the thoracic aorta was removed and placed in a Petri dish containing Krebs-Henseleit solution (KHS) ([mM]: NaCl, 118.2; KCl, 4.7; MgSO4·7; H2O, 1.2; CaCl2, 2.5; KH2PO4, 1.2; NaHCO3, 25; glucose, 11.1). After cleaning the surrounding tissues, 3–4-mm-long aortic rings were obtained from each animal and allocated to the following study groups randomly: control (C), ischaemia (I), hypoxia (H), ischaemia-reperfusion (IR), and hypoxia-reperfusion (HR) groups. Aortic rings allocated to the control group were directly placed in organ baths, and the experimental protocol was implemented. The study groups and experimental procedures were summarized in Figure 1.
Protocol for hypoxia, ischaemia, and reperfusion
Aortic rings allocated to the ischaemia, hypoxia, ischaemia-reperfusion, and hypoxia-reperfusion groups were stored at 4°C for 24 h in cold saline-containing tubes. For ischaemic preservation, tubes were previously gassed with nitrogen (N2) to remove oxygen from the solution. For hypoxic preservation, tubes had been stirred to obtain equilibrium with atmospheric oxygen. After 24 h, aortic rings were placed into the organ baths, and oxygen levels in the preservation tubes were measured via an oxygen sensor electrode (WPI ISO-OXY-2, MAY, Turkey). The aortic rings in reperfusion groups were incubated with 200 μM sodium hypochlorite (NaOCl) for 30 min in organ baths, to mimic reactive oxygen species generation and endothelial damage, which occur during reperfusion.
Experimental protocol for isolated organ bath
The aortic rings were mounted on 2 stainless steel hooks in organ baths containing 10 ml of KHS and continuously aerated with 95% O2 and 5% CO2 at 37.4°C. One of the hooks was fixed vertically to the organ sling, and the other one was connected to an isometric force-displacement transducer (FDT05, Commat Ltd., Turkey), which transmits isometric tension changes to a computerized physiological data acquisition and recording system (BIOPAC MP100, Commat Ltd.). The aortic rings were equilibrated for 1 h at 1 g resting tension before any experimental intervention. During this period, baths were rinsed with KHS, which was changed every 15 min to prevent metabolite accumulation, and the tension was adjusted periodically to the desired level. After the equilibration period, maximum contractile responses to 80 mM potassium chloride (KCl) were obtained to evaluate vascular smooth muscle contractility, and aortic rings were washed until the resting tension was again obtained. Subsequently, the aortic rings were contracted with cumulative concentrations of a1-adrenergic receptor agonist phenylephrine (PE, 10–11–10–5 M), and the concentration of PE that evoked submaximal (at least 80% of its maximum) contractile response was calculated for each ring. Then, cumulative concentrations of acetylcholine (ACh, 10–11–10–5 M), an endothelium-dependent vasorelaxant, were added to the aortic rings precontracted with the submaximal concentration of the PE. To test the endothelium-independent relaxation response of smooth muscle, responses to cumulative concentrations of sodium nitroprusside (SNP, 10–12–10–7 M), a direct nitric oxide donor, were obtained on the aortic rings precontracted with the submaximal concentration of PE.
Statistical analysis
The experimental unit was the “rat” in our study. The required sample size was calculated as 9 rats to determine the large effect size (Cohen’s d = 1.5) with a power of 80% at a significance level of 0.05 in paired study groups. Twelve rats were included in the study, taking account for any losses that might occur during the experiment. Out of 5 aortic rings obtained from each rat, at least 2 were unresponsive to KCl in 3 rats, thus data from 9 rats were included in statistical analysis.
PE contractions were expressed as a percentage of the maximum KCl contraction, while ACh and SNP relaxations were expressed as a percentage of the submaximal PE contraction response. The concentration inducing half-maximal response (EC50), the measure of sensitivity (–logEC50, pD2), and the maximum response (Rmax) for PE, ACh, or SNP concentration-response curves were calculated with nonlinear regression and given with 95% confidence intervals (CI). The differences between study groups in maximum KCl contraction and best-fit parameters were tested using one-way analysis of variance (ANOVA) followed by Holm-Sidak’s post hoc test for multiple comparisons for normally distributed data. The difference between study groups for concentration-response curves was assessed with 2-way repeated-measures ANOVA. Pairwise comparison of curves was performed with extra sum-of-squares F test in nonlinear regression analysis. The normal distribution was tested using the Anderson-Darling or D’Agostino and Pearson normality tests.
Statistical analyses were performed using GraphPad Prism 5.0 software (GraphPad Software Inc., San Diego, CA, USA), the significance limit was determined as p < 0.05.
Results
The oxygen concentration measured by the oxygen sensor electrode was 6% O2 in ischaemic preservation tubes and 22% O2 in hypoxic preservation tubes.
KCl- and PE-induced vascular contractions
KCl (80 mM) induced 1614.0 ±82.9 mg isometric contractions in the aortic rings of the Control group (n = 9). One-way ANOVA showed a significant difference among study groups in terms of KCl-induced contractions (p = 0.007, Figure 2 A). The response to KCl was impaired in all experimental groups, but did not significantly differ between IR, HR, I, and H groups in post-hoc analysis (Figure 2 A).
Figure 2
A – KCl (80 mM)-induced isometric contractions of the aortic rings in the study groups, which were given as the mean ± standard error of the mean. p = 0.007 (one-way ANOVA). ***p < 0.0001, *p < 0.05 (post-hoc multiple comparison tests). B – concentrationresponse curves for phenylephrine-induced contractions fitted with nonlinear regression. Each point represents the mean ± standard error of the mean. p = 0.020 (2-way ANOVA). ***p < 0.0001 for pairwise comparison of curves in nonlinear regression analysis
C – control, H – hypoxia, HR – hypoxia-reperfusion, I – ischaemia, IR – ischaemia-reperfusion, KCl – potassium chloride.
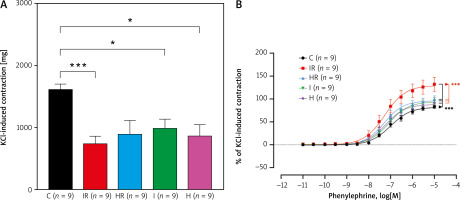
PE induced concentration-dependent contractions in all groups (n = 9 for each group) (Figure 2 B). Two-way ANOVA identified a statistical difference between study groups in PE concentration-response curves (p = 0.020). Comparison of concentration-response curves in the nonlinear regression model indicates that curves of IR, HR, I, and H were significantly different from the control curve (p < 0.0001 for all). Additionally, the curve of IR was significantly different from the curves HR, I, and H (p < 0.0001 for all). However, there was no significant difference between the curves of the HR and I groups (p = 0.1653), HR and H groups (p = 0.1595), and I and H groups (p = 0.7210) (Figure 2 B). In the control group, the maximum PE contraction was 82.4% of KCl contraction (95% CI: 74.6–90.3%), and pD2 was 7.0 (95% CI: 6.9–7.1) (Table I). While pD2 did not differ significantly between the groups, maximum PE response was significantly enhanced only in the IR group compared to the rest of the study groups (p < 0.0001, Table I).
Table I
Best-fit values (95% confidence interval) for pD2 and the maximum response of nonlinear concentration-response curves of PE, ACh, and SNP in the study groups
Variable | C (n = 9) | IR (n = 9) | HR (n = 9) | I (n = 9) | H (n = 9) | P–value |
---|---|---|---|---|---|---|
Concentration-response curve for PE: | ||||||
–logEC50 (pD2) | 7.0 (6.9–7.1) | 7.3 (6.9–7.5) | 7.3 (7.1–7.5) | 7.1 (6.9–7.2) | 7.2 (7.0–7.4) | 0.1562 |
Maximum response (%) | 82.4 (74.6–90.3) | 131.2 (96.9–165.4) | 99.5 (83.3–115.7) | 93.9 (78.5–109.3) | 90.1 (75.7–104.5) | < 0.0001a |
Concentration-response curve for Ach: | ||||||
–logEC50 (pD2) | 7.2 (6.8–7.7) | 5.1 (2.5–7.7) | 4.3 (0.7–8.0) | 6.1 (4.4–7.8) | 6.7 (6.2–7.2) | 0.7239 |
Maximum response (%) | 73.4 (70.2–76.8) | 23.7 (14.4–33.1) | 34.2 (23.7–44.7) | 52.7 (30.3–75.0) | 59.3 (45.8–72.7) | 0.0002b |
Concentration-response curve for SNP: | ||||||
–logEC50 (pD2) | 8.3 (7.4–8.6) | 8.3 (8.1–8.4) | 8.1 (7.5–8.3) | 8.1 (7.3–8.4) | 8.1 (7.6–8.3) | 0.7889 |
Maximum response (%) | 95.2 (90.5–99.8) | 105.2 (97.1–113.4) | 106.2 (88.4–124.0) | 97.6 (90.9–104.3) | 90.9 (76.9–105.0) | 0.9079 |
Endothelial-dependent and -independent vascular relaxations
ACh induced concentration-dependent relaxations in the aortic rings, with significant differences between the groups (Figure 3 A, p = 0.0011). In nonlinear logistic regression analysis, concentration-response curves for ACh relaxations were significantly impaired in all experimental groups compared to the control group (p < 0.0001 for all, Figure 3 A). This impairment was minimum in the non-reperfusion groups (I and H) and maximum in the reperfusion groups (IR and HR) (p < 0.0001 for all, Figure 3 A). It was also noted that there was a significant difference between the ACh concentration-response curves of the IR and HR groups (p < 0.0001), and the endothelium-dependent vascular relaxation responses were largely lost in the IR group (Figure 3 A). The ACh concentration-response curves of the I and H groups were not significantly different (p = 0.4314). Analysis of best-fit values showed that the pD2, a measure of the sensitivity of aortic rings to ACh, was not significantly different across study groups (Table I, p = 0.7239). However, maximum relaxations obtained by ACh were significantly impaired in the aortic rings of the IR and HR groups compared to the control group (p = 0.0002), without any difference between the IR and HR groups (Table I). The aortic rings in I and H groups – non-reperfusion groups – induced maximum ACh relaxations (Figure 3 A, Table I).
Figure 3
A – Concentration-response curves for acetylcholine-induced relaxations fitted with nonlinear regression. p = 0.0011 (2-way ANOVA). ***p < 0.0001 for pairwise comparison of curves in nonlinear regression analysis. B – Concentration-response curves for sodium nitroprusside-induced relaxations fitted with nonlinear regression. p = 0.1258 (2-way ANOVA). Each point represents the mean ± standard error of the mean
C – control, H – hypoxia, HR – hypoxia-reperfusion, I – ischaemia, IR – ischaemia-reperfusion, PE – phenylephrine.
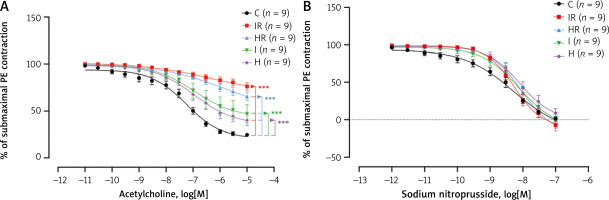
There was no difference between the study groups in terms of endothelial-independent relaxations induced with SNP (Figure 3 B, p = 0.1258) and in pD2 and the maximum relaxation of SNP concentration-response curves (p = 0.7889 and p = 0.9079, respectively; Table I).
Discussion
This study primarily showed that cold hypoxia (22% O2) for 24 h followed by reperfusion is sufficient to induce vascular contractile dysfunction of rat isolated thoracic aortic rings; however, ischaemia (6% O2) followed by reperfusion provided the most evident dysfunction of both the endothelium and vascular smooth muscle. These findings are noteworthy because they present a re-evaluation of a common in vitro model of vascular graft I/R injury in aged animals by comparing the chemically confirmed hypoxic and ischaemic conditions. Depending on our reappraisal, the cold I/R model is suitable for in vitro studies to investigate the pathophysiology of vascular graft failure and graft preservation options.
In the literature, various hypoxia/ischaemia protocols were implanted, such as gassing the Krebs solution with 95% N2/5% CO2 for 15–120 min without any cold preservation period [14, 15], cold storage at 4°C for 24 h after gassing with N2 [9–12], and cold storage at 4°C for 24 h without pretreatment with N2 [16, 17]. Reperfusion injury is usually induced by exposure of tissue to 200 µM hypochlorite or 200 µM peroxynitrite, external oxidant sources, or reoxygenation of organ bath after hypoxia [9–11, 15–18].
In our experimental setup, we investigated cold ischaemic and hypoxic preservation for 24 h followed by NaOCl-induced reperfusion injury to evaluate the effects of different oxygen levels on in vitro vascular responses of aged rats. We found that the contractile response to high concentration of KCl was impaired in all study groups – impairment in the HR group was remarkable but statistically insignificant (C vs. HR, p = 0.0635). The decreased contractile response to KCl indicates that depolarization-induced vascular smooth muscle contractility is impaired by ischaemia or hypoxia with or without reperfusion. This impairment is explained by disruption of KCl-induced calcium sensitization or calcium ion influx from extracellular medium during long-term cold ischaemic or hypoxic conditions [19–21].
We also showed that 24-hour ischaemic or hypoxic cold preservation increased the sensitivity of vascular tissue to a1-adrenergic receptor agonist PE causing increased contractile response. The supraphysiologic vasoconstriction response is most prominent in the aortic rings of the IR group. This is in line with previous studies reporting enhanced contractile response to PE after in vitro I/R injury, it is and explained by the deterioration of the vascular relaxation response and an unbalanced increase in contraction [9–12]. The fact that the increase in phenylephrine response was highest in the IR group is evidence that in vitro cold ischaemia and subsequent reperfusion is the suitable representative model of graft endothelial damage. Endothelial dysfunction plays an important role in graft failure and should be the target of vascular graft preservation research.
The ACh-induced endothelium-dependent vascular smooth muscle relaxation is widely used in vitro protocol to test endothelial function [9–11, 15–18]. Our findings confirm previous studies that 24-hour cold ischaemic preservation followed by reperfusion impairs endothelial vasorelaxant function [9, 21, 22]. When compared to the control group, endothelium-dependent relaxation responses of the reperfused aortic rings from both IR and HR groups were decreased significantly. The maximum impairment of endothelium-dependent relaxation was recorded in the IR group, a finding which supports that in vitro cold ischaemia and subsequent reperfusion is the suitable representative model of graft endothelial damage. It is worth mentioning that endothelium-dependent relaxation responses of the aortic rings from not reperfused groups, H and I, were also decreased. This may indicate that in addition to reperfusion injury, the low oxygen levels of vascular graft preservation solutions disrupt the endothelial integrity. Our findings from the SNP-induced endothelium-independent vascular relaxations show that the smooth muscle function remained intact in hypoxic and ischaemic conditions, indicating that the vascular endothelium is the main target of I/R injury.
The present study is a re-evaluation of the in vitro vascular ischaemia reperfusion model, which is a common but not-standardized model for I/R injury of graft vessels. This is the first study to biochemically confirm the ischaemic and hypoxic conditions applied in the model. Additionally, it has been studied on aged animals to increase the translational value of the model. However, there are also limitations that should be noted. One limitation of the study is that hypoxia or ischaemia was only applied for 24 h, and the different durations of hypoxia/ischaemia were not evaluated. In addition, because the aim of the study was to compare hypoxic and ischaemic conditions, we only applied a standard reperfusion protocol, rather than comparing different reperfusion protocols. Furthermore, considering that reperfusion cannot occur without ischaemia in clinical conditions, a separate reperfusion group was not formed. Additionally, although we studied aged animals considering increased vascular damage in elderly, only male animals were included, which makes it difficult to extrapolate our findings to the female population. Another limitation of the study is that the aortic rings were not weighed, and vascular smooth muscle responses were not standardized for tissue weight. For this limitation, we prepared the vascular rings in the same size and expressed the contraction and relaxation responses as percentages. However, a complete standardization would have been achieved if the KCl contraction given in mg was corrected according to tissue weight. Finally, the physiological, cellular, and molecular mechanisms involved in the vascular dysfunction caused by hypoxia/ischaemia and reperfusion were not evaluated. Because our focus in the present study was only to compare ischaemia and hypoxia to find the best in vitro model representing the vascular ischaemia-reperfusion injury, we did not add any antagonists to the organ bath and did not perform mechanistic evaluation. Further research should be conducted to determine the mechanisms of ischaemia and reperfusion injury that lead to vascular smooth muscle and endothelial dysfunction, as well as agents that target these mechanisms.