Introduction
According to the electronic press release of the World Health Organization (WHO) on June 11, 2021, the major cause of global death is cardiovascular diseases (CVDs). Approximately 32% of all global deaths (17.9 million) were caused by CVDs in 2019, which includes 85% of deaths due to cardiac stroke and heart attack. In the noncommunicable diseases category, an estimated 38% of premature deaths occurred in 2019 from CVDs in people who were under 70 years old [1]. Therefore, it is necessary for researchers to develop clinical interventions for cardioprotection and prevention of cardiac diseases. Already a significant number of studies have shown that nicotinamide adenine dinucleotide (NAD+) plays a pivotal role as a co-substrate for multiple enzymes that are directly or indirectly involved in several signal-transduction pathways effective in CVDs. There are two bio-mechanisms for NAD synthesis in mammals: i) In the tryptophan-based de novo pathway, quinolinic acid (QA) is synthesized from food-based tryptophan. The QA is then transformed into nicotinic acid mononucleotide (NaMN) in the presence of quinoline phosphoribosyl transferase (QAPRT). In the next step, NaMN is utilized to form nicotinic acid adenine dinucleotide (NaAD) by nicotinic acid mononucleotide adenyl transferase (NaMNAT). The NaAD is then amidated to NAD+ by NAD synthase (NaDS). ii) The second is the nicotinamide (NAM), nicotinamide riboside (NR) or nicotinamide mononucleotide (NMN), and nicotinic acid (NA) based salvage pathway [2–4]. NAM, NR, and NA are also well known as three different forms of vitamin B3 used supplementarily to enhance NAD+ levels inside cells [5–7]. In the salvage pathway, NAD can be produced by a) nicotinamide phosphoribosyl transferase (NAMPT) through nicotinate mononucleotide (NAMN) from NA; b) nicotinate phosphoribosyl transferase (NAPRT) produces NAD through the complex process of the Preiss-Handler pathway where nicotinic acid mononucleotide (NAMN) is synthesized from NA then conjugated with ATP to get NAD; and, c) nicotinamide riboside kinases (NRKs) produce NAD by converting NR to NMN and nicotinamide mononucleotide adenyl transferase (NMNAT) [8, 9]. Figure 1 presents the details of the NAD synthesis process in different pathways.
Figure 1
Synthesis pathway of nicotinamide adenine dinucleotide (NAD) in mammals: de novo pathway demonstrates NAD formation from tryptophan and the salvage pathway produces NAD with the help of three main enzymes: nicotinamide phosphoribosyl transferase (NAMPT), nicotinate phosphoribosyl transferase (NAPRT) and nicotinamide riboside kinases (NRK); QAPRT – quinolinate phosphoribosyl transferase, NMN – nicotinamide mononucleotide; NMNAT – nicotinamide mononucleotide adenyl transferase
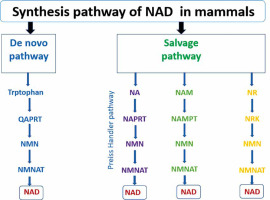
The latest research findings showed that treatment with a well-known NAMPT activator pharmaceutical (P7C3) significantly improved the synthesis of p-AKT (protein kinase B), p-eNOS (endothelial nitric-oxide synthase), and Beclin 1; in addition, there were decreases of the important cardiac health indicators troponin I, lactate dehydrogenase (LDH) release, which is secreted more in poor heart condition, and reduced infarct size in diabetic mice hearts, which plays an important role as a cardio protector [10]. Another research group demonstrated that NAPRT plays a vital role in protecting cells from oxidative stress by increasing NAD production from NA [11]. It was reported that NRK significantly contributes to cardioprotection following heart failure after ischemia [12]. Several studies have demonstrated NAD activity in different cardiac conditions, which established NAMPT, NAPRT, and NRK as rate-limiting enzymes in the salvage pathway. In this comparative study, we mainly investigated how three main rate-limiting enzymes – NAMPT, NAPRT, and NRK – play an important role in NAD+ synthesis through salvage pathways to maintain cardiac health and well-being.
Association of NAD+ with cardiac health
Since the discovery of nicotinamide adenine dinucleotide (NAD) in 1906 by the British biochemist Arthur Harden [13], it has become a hot topic for researchers working in this domain. NAD is a pyridine nucleotide that works as a main component in a variety of cells to catabolize the fuel substrate [14]. The internally integrated compound NAD+ works as an activity modulator in target-specific cellular metabolic processes such as modulation of the glycolysis cycle in the cytoplasm and the tricarboxylic acid (TCA) cycle in the mitochondria [15]. A pair of NAD+ molecules are required for a single molecule of glucose to synthesize pyruvate in the cytoplasmic glycolysis process followed by acetyl-CoA (coenzyme A) and oxidation of pyruvate. Another pathway to produce acetyl-CoA is β oxidation of fatty acid through NAD+ to NADH formation. The mitochondrial TCA cycle then utilizes the acetyl-CoA to form multiple NADH from NAD+ molecules. Oxidative phosphorylation at the mitochondrial membrane again converts NADH molecules into NAD+, leading to electron production and a change of cellular barrier potentials which especially plays a vital role in cardiac action potential generation in depolarization and repolarization phases. Recent research findings demonstrated that treatment with NAM can restore cardiac NAD+ by increasing the production of lactate, glycolysis, and ATP following cardiac ischemia, which helps in contractile recovery with an increasing rate of survival in a mouse model of cardiac arrest [16]. There have been several published studies on the relationship between heart diseases and the role of NAD+ in this action. Also, the role of NAD+ synthesizing enzymes in the salvage pathways is intensively studied. Based on the literature to date, the key role of NAD+ in cardiac health is to control cardiac energy metabolism, help to recover from coronary heart disease, cardiac arrhythmia, vascular homeostasis-related cardiovascular disease, anti-inflammation processes of the heart, heart failure, ischemia/reperfusion injury, cardiac dysfunction, fibrosis, rapid LV chamber dilatation, and anti-aging effects on the heart [14, 17, 18].
Table I is a summary of the most recently published work showing the role of NAD+ in cardiac health. The main NAD synthesizing regulators in the salvage pathway are also highly studied to understand the actual role of NAMPT, NAPRT, or NRK [19–35].
Table I
Role of nicotinamide adenine dinucleotide (NAD+) in cardiac health
Cardiac condition | Key findings | Ref. |
---|---|---|
Cardiometabolic disorder (heart failure) | Role of NAD+ in pathophysiological processes and therapeutic potential in cardiac diseases | [19, 20] |
Chronic heart failure | NAD+ can alleviate chronic heart failure via the mitochondrial oxidation-reduction (redox) state mechanism | [21] |
Post-resuscitation myocardial dysfunction | Exogenous NAD+ treatment attenuated post-resuscitation myocardial dysfunction | [22] |
Cardiovascular issues | NAD+ helps protect against heart failure | [23] |
Heart failure (HF) | Raising NAD+ levels may represent a novel HF treatment | [24] |
Myocardial ischemia/reperfusion injury | NAD+/ NADH regulator GSK-3β protects against myocardial ischemia/reperfusion injury | [25] |
Systolic blood pressure | NAD supplementation may improve decreasing systolic blood pressure | [26] |
Maternal vascular dysfunction during a high-risk period | Vascular NAD+ depletion may contribute to functional impairment | [27] |
Cardiac health | NAD+ improves cardiac health | [28] |
Cardiomyocytes | Metoprolol ameliorated the condition of cardiomyocytes by affecting NAD+ | [29] |
Hypertrophic cardiomyopathy | NAD severely reduced in hypertrophic cardiomyopathy compared with control hearts | [30] |
Cardiometabolic complications | SIRT levels may be involved in cardiometabolic complications which are directly linked with NAD | [31] |
Vascular remodeling | NAD+ modulation shows promise in the vascular remodeling of endothelial cells | [32] |
Cardiac complications in patients with diabetes | Promotion of mitochondrial fusion through increased NAD+ synthesis via oral supplementation of NR as a potential strategy for delaying cardiac complications in patients with diabetes | [33] |
Cardiolipin deficiency | Supplementation with nicotinamide mononucleotide (NMN) attenuates mitochondrial dysfunction associated with cardiolipin deficiency | [34] |
Cardiovascular disorders | Role of nicotinamide adenine dinucleotide phosphate (NADPH) in cardiac diseases | [35] |
The role of NAMPT in cardiac health
One of the main enzymes of NAD+ synthesis in the salvage pathway of mammals is nicotinamide phosphoribosyl transferase (NAMPT) encoded by the NAMPT gene in the human body. Intracellular NAMPT (iNAMPT) works as a rate-limiting enzyme in the nicotinamide adenine dinucleotide (NAD+) salvage to form nicotinamide mononucleotide (NMN) from nicotinamide. Extracellular NAMPT (eNAMPT) has emerged as a cytokine in pre-β-cell colony-enhancing factor (PBEF) that helps the maturation of β cells by activating Toll-like receptor 4 (TLR4) [36]. It was reported that pharmaceutically activated NAMPT in a diabetic mouse model decreased the NADH/NAD+ ratio and improved overall cardiac function by improving p-AKT, p-eNOS, and Beclin 1 expression [37]. Overexpression of NAMPT can increase NAD+ synthesis and ATP levels in cardiomyocytes to protect against myocardial injury due to in vitro myocardial ischemia and reperfusion [38]. On the other hand, research on knockout mice and a murine model showed that cardiac-specific NAMPT overexpression can cause pressure overload-induced heart failure by reducing protein acetylation, mutation of specific genes, and alteration in mitochondrial energy production [39]. This finding indicated the specific role of NAMPT in improvement of cardiac function in the damaged heart. At the same time, cardiac-specific NAMPT overexpression showed important changes in cardiac hemostasis and heart function. Research revealed that visfatin, a well-known paracrine in the cardiovascular system, has nicotinamide phosphoribosyltransferase (NAMPT) activity to maintain cardiac function [40]. Treatment with NAMPT and NAD+ improves the mitochondrial reactive oxygen species (ROS) concentration and reduces gap junction (a path of electrical propagation) activity in cardiac endothelial cells (CEC) of diabetic mice [41]. Another study revealed that the development of pulmonary arterial hypertension (PAH) in a mouse model was directly linked to nicotinamide phosphoribosyl transferase (NAMPT) [42]. In hypertrophic cardiomyopathy (HCM) a decreased level of NAMPT was observed [43]. Cardiac toxicity is a common problem where supplementation of selenium showed beneficial effects against arsenic trioxide-induced cardiotoxicity by reducing oxidative stress and inflammation through overexpression of NAMPT and NAD+ levels [44]. A handful of publications have described the positive role of NAMPT in cardio-protective actions against several complexities. Figure 2 at the inset demonstrates the effect of NAMPT on cardiac health.
The biological significance of NAPRT in cardiac health
The second main rate-limiting enzyme in the salvage pathway for NAD synthesis is nicotinate phosphoribosyl transferase (NAPRT). Genetically the chromosomal location of the NAPRT gene in the human body is 8q24.3, containing 12 exons. The presence of intracellular NAPRT is very similar to NAMPT and situated in the nucleus as well as in the cytoplasm like the presence of NAMPT, but absent in the mitochondria [45, 46]. The biosynthesis of NAD in humans is controlled by dimeric protein-based phosphoribosyl transferase (NAPRT, Type II) with the combined effort of quinoline phosphoribosyl transferase (QPRT) and NAMPT. It was revealed that NAPRT activity is not influenced by NAD as in the case of NAMPT [47]. The NAD regulation by NAPRT is specific to tissue and certain conditions [48]. The latest research on tumor cells revealed that the NAMPT activity highly depends on NAPRT expression [49]. Interestingly, NAPRT is strongly activated by phosphate [50]. Based on the author’s knowledge, there is no available literature to date with direct evidence of NAPRT’s influence on cardiac health. But the research on other applications including cancer [51], sepsis/septic shock [52], and treatment in prostate, ovarian, colorectal, and pancreatic disease [53–55] showed great potential. However, NAPRT appears as a driver in inflammatory processes to control the activation of macrophages necessary to recover from acute inflammatory diseases such as sepsis and septic shock [56]. In this context, supplementation-based activation of NAPRT boosts NAD levels in cultured cells to decrease cytotoxicity induced by oxidative stress [57]. Therefore, it may be a prospective research area to study the direct/indirect influence of NAPRT on cardiovascular activity especially in CVD to improve cardiac health. Figure 3 shows how NAPRT supplementation improves NAD levels and helps to control cardiac inflammation caused by oxidative stress, toxicity, etc.
Nicotinamide riboside kinase (NRK) and its role in cardiac health
In 2004, it was found that NAD+ can also be synthesized by NRK, which was initially detected in yeast as nicotinamide riboside kinase 1 (NRK1) followed by human homologs NRK1 and NRK2 [58, 59]. NRK1 activity is more prone to occur in mammalian tissues; on the other hand, NRK2 is specific to muscle and more significantly predominant in skeletal muscle than cardiac [12, 60]. Findings from research on wild-type (WT) mice showed that the modulation effect of NAD+ by NR and NMN supplementation is ineffective if the NRK enzyme is absent in the tissue whereas other enzymes for the NAD+ synthesis pathway remain active in WT mice [61, 62]. A recent research communication reported that NR can significantly protect NAD+ synthesis by pharmaceutically influencing the NAMPT effect in cardiomyocytes [63]. It also stated that food supplementation of NR can attenuate HF mice by stabilizing myocardial NAD+ levels in the failing heart in dilated cardiomyopathy (DCM), and partially after transverse aorta constriction (TAC) [64]. Research showed the crucial role of NRK-2 in heart failure caused by injury due to ischemia and the deficiency of NRK-2 accelerates the spread of scarring after MI, faster dilatation of the LV chamber, cardiac dysfunction, and fibrosis [23]. The addition of NRK supplementation has become the most popular technique to affect NAD+ levels in different health conditions including CVDs. The cardioprotective role of NR supplementation is shown in Figure 4.
The combined effect of NAMPT and NAPRT
There is no doubt that NAMPT and NAPRT actively and effectively work for NAD synthesis, which is the key mechanism in the cellular pathway. It is now evident that the influence of intracellular NAD level employing treatment with NAD activators in elderly mice has shown versatile anti-aging effects such as reversing age-related complexities in different organs, including the eye [8], skeletal muscle [20], and brain [65]. Recent research demonstrated that short-term administration of NMN produced prolific results in a diverse pathophysiological state in aging problems, improving the functional activity of skeletal muscle by energizing it [66]. The NAD effect on neuronal stem cell protection and lengthening the active life span of mice is shown in the latest research [67]. Enhanced activity of mitochondria is observed in the skeletal muscle under the hyperlipidemia interaction in type 2 diabetic patients by the effect of acipimox, a well-known NAD booster [68]. Increasing evidence has revealed the combined effect of NAMPT and NAPRT in physiological and pathophysiological roles [69, 70]. The combined role of NAMPT and NAPRT in inflammation was also observed [49]. Another study on colorectal cancer (CRC) showed that the duration of disease-free survival time and overall survival time from CRC is indicated by NAMPT or NAPRT expression and the excessive level of NAMPT/NAPRT indicates the improper prognosis of patients with CRC in a distinct mechanism [52]. There are several research groups already working to find out the combined effect of NAMPT and NAPRT in different clinical domains, but to date, no conclusive data are available related to the combined effect on cardiovascular application. According to the authors’ point of view, the combined study of NAMPT, and NAPRT might bring a crucial outcome in the field of clinical cardiology and other fields of medical interventions. Figure 5 demonstrates the added advantages of controlling NAD+ levels utilizing the combined effect of NAMPT and NAPRT.
The combined effect of NAMPT, NAPRT, and NRK
Maintaining a healthy level of NAD+ has great clinical significance; alteration of the normal level predicts different health complications [71]. It is proved that NAD+ supplements can increase lifespan in yeast and worms [72, 73]. Several studies have revealed that NAD+ precursors are potential candidates for clinical applications such as fatty liver disease, kidney injury, hearing loss, a neurodegenerative disorder, aging-related problems, and metabolic dysfunction [74]. The main reasons for considering NAD+ levels as intra-cellular activity indicators are that a) it works as a mandatory redox co-factor in multiple cellular reactions, and b) it can be divided into a wide range of different enzyme families to be utilized as a substrate for their main catalytic activities [75]. The latest finding demonstrated NR as an NAD+ booster in human applications [76, 77]. Data show that enhanced NAD+ levels by supplementation inhibited myocardial fibrosis [22]. Heart damage due to ischemia-reperfusion injury requires high NAD+ to control the infarct size and prevent cardiac injury. The causes of CVDs such as cardiomyopathy [78], atherosclerosis [79], and heart failure [80] are also associated with metabolic disorders. Boosting NAD+ levels through metabolism may help to improve these unwanted critical heart conditions. It was recently found that the addition of NR in the form of a supplement can prevent heart failure by enhancing citrate and acetyl-CoA through the glycolysis pathway [12]. Recent findings demonstrated the combined effect and role of NAMPT and NAPRT in inflammation [81, 82]. A few other studies have demonstrated the combined effect of NAMPT and NAPRT. However, the combined effect of three main NAD+ regulators in the salvage pathway has not been demonstrated yet, though the potential effect of this combined application may be more prolific in targets specific for different disease conditions. The inset in Figure 6 shows how three rate-limiting enzymes can provide greater health benefits and enhance control of health parameters to protect against disease conditions.
Prospects of enzyme-activated NAD+ synthesis in CVD
There is no doubt that NAD+ plays a leading role in cardiovascular health and heart activity monitoring through intra- or extracellular pathways. It is known that NAD+ is heterogeneously distributed in the cell [83–86] and within the subcellular tissue, NAD+ distribution is very uneven [87]. The NAD+ permeability in the subcellular organelles also varies. Though NAD+ can easily be translocated through the nucleus and cytoplasm of the cell, the rate of translocation is different in the mitochondria than in the nucleus and cytoplasm [88]. A recent study by Luongo et al. was successful in detecting SLC25A51, which is an NAD+ transporter inside mammalian mitochondria [89] and may work as a missing link to understand the complex mechanism of NAD+ uptake into the mitochondria. On the other hand, the activity and control of NAD+ synthesizing enzymes at the subcellular level also differ. Interestingly, the major NAD+ synthesizing enzymes nicotinamide riboside kinase 1 (NRK1), nicotinamide riboside kinase 2 (NRK2) [90], and nicotinate phosphoribosyl transferase (NAPRT) are identified in the cytosol only [91]. In contrast, NMNAT in different isoforms is present in the cytosol, nucleus, mitochondria, and Golgi apparatus [92]. In association, nicotinamide phosphoribosyl transferase (NAMPT) is present in the cytosol, nucleus, and mitochondria [93], with NAM as a common precursor of NAD+. The way of NAD+ production and/or the supplementation method to modulate or demodulate NAD level might change the subcellular distribution of NAD+ inside the cell. Significantly, the ever-increasing result of NAD+ modulation by different pharmaceuticals or activators in salvage pathway has shown a wide range of applications for cardiac protection and treatment of CVDs including atherosclerosis, coronary heart disease, hypertension, arrhythmias, and heart failure [94]. Based on the concept of NAD+, there are two main possibilities in different physiological conditions: either an increase of NAD+ or a decrease in the level of NAD+, which can be controlled by treating with NAD+ activators and inhibitors, respectively. The principal mechanism of NAD+ activators/inhibitors is associated with three main enzymes: NAMPT, NAPRT, and NRK. The main clinical research options available to date are to either modulate/demodulate NAD+ utilizing an NAMPT/NAPRT activator/inhibitor or NRK supplementation. A few recent studies have also demonstrated the combined physiological effect of two enzymes at a time. However, to date there are no available data from studies where NAMPT, NAPRT, and NRK were each studied for a particular application. In the case of cardiac applications NAD+ activators/inhibitors are becoming established as primary options for CVD management. Individual NAD+ modulators through NAMPT/NAPRT activators or NRK supplementation have already shown prolific treatment options in different clinical applications including CVDs. Therefore, the prospect of NAD+ alteration as a combined study may become the most relevant and prolific step in cardiovascular applications.
Conclusions
The role of NAD+ in cardiac health is well known, with NAMPT, NAPRT, and NRK being revealed as the main controllers in NAD+ synthesis in the salvage pathway. Various clinical trials have revealed that modulation or demodulation of NAD+ level by supplementing any one of the activators or a combination of two can improve cardiac health condition by improving the overall NAD+/NADH ratio towards the normal level, which ultimately improves the health condition from the disease state and promotes faster recovery. This paper is a commentary on these three main NAD+ activators in association with cardiac health and the future scope of work to alter NAD+ levels by changing these three enzymes together or individually, which may provide better health effects.