Summary
There are conflicting data on a potential relationship between transcoronary stem cell transfer and progression of atherosclerosis. Some, mostly small-size and poorly controlled, clinical studies hypothesized that transcoronary-administered stem cells might stimulate atherosclerosis whereas some other (mostly basic research) investigations suggest several mechanisms whereby different types of stem cells might inhibit progression of atherosclerosis. Using a range of classic and novel imaging techniques we performed a longitudinal investigation in consecutive patients receiving transcoronary stem cell administration in the sub-acute phase of myocardial infarction. We found no stimulatory effect of transcoronary stem cell transfer on coronary atherosclerosis; a finding that is reassuring for clinical studies employing the transcoronary avenue as the route of choice for delivery of stem cell-based therapies to compromised myocardium. By intravascular ultrasound there was a moderate reduction in stenosis severity at 12-months and the lesions maintained low-risk lesion phenotypes on virtual histology assessment. Whether this apparent benefit is related to pharamacologic therapy and/or stem cell transfer requires further elucidation.
Introduction
Despite unquestionable progress in pharmaco- and device therapies of atherosclerosis and consequences of atherosclerosis-elicited ischaemic events, the burden of ischaemic heart disease (including ischaemic heart failure as a result of myocardial infarction(s)) is anticipated to further increase in the next 2 decades [1, 2]. Cell-based therapies aimed to stimulate myocardial repair and regeneration play an important role in current strategic agenda for cardiovascular research [1, 3]. Presently, cardiovascular cell-based therapies are undergoing some critical refinements [4–6]. Nevertheless, safety and minimization of any potential adverse effects remain as fundamental considerations in both early clinical studies and later-stage trials [4, 7].
The transcoronary route has been the principal avenue for stem/progenitor cell delivery to the myocardium, particularly in the setting of acute myocardial infarction (AMI) [8–10], using either a conventional “stop-flow” technique [9] or, more recently, physiologic perfusion techniques of cell delivery [11–14]. Some, mostly small-size and uncontrolled, studies hypothesized that transcoronary administration of stem cells might stimulate progression of atherosclerosis [15, 16], raising a potential concern for clinical trials using transcoronary cell delivery.
Aim
We prospectively evaluated, using conventional (quantitative coronary angiography (QCA), intravascular ultrasound (IVUS) and traditional VH-IVUS) and novel (quantitative virtual histology – qVH) imaging tools, evolution of infarct-related artery (IRA) atherosclerosis following transcoronary stem cell transfer via IRA.
Material and methods
We performed an investigator-initiated substudy of the Myocardial Regeneration by Intracoronary Infusion of Selected Population of Stem Cells in Acute Myocardial Infarction (REGENT) Trial (NCT00316381) [8]; it included the REGENT patients receiving autologous CD34+CXCR4+ cells in our center. Detailed inclusion/exclusion criteria were provided previously [8]. In short, inclusion criteria were as follows: anterior (AMI), successful primary percutaneous intervention (pPCI) with stent implantation in the proximal segment of IRA (left anterior descending coronary artery – LAD) within 12 h after the symptom onset, reduced left-ventricular ejection fraction (LVEF) ≤ 40%, and age 18–75 years. Fundamental exclusion criteria were prior MI, presence of significant stenoses in other coronary vessels requiring revascularization, past or present malignancy, or contraindications to magnetic resonance imaging (cMRI). All patients received medications as recommended by current guidelines.
On a median day 7 post stent-assisted pPCI, infusion CD34+ CXCR4+ selected autologous bone marrow stem cells was performed via the artery supplying the region of the myocardium affected by the infarction (LAD). Stem cells were administered through a stemcell delivery-dedicated perfusion catheter [11], positioned immediately distal to the stent in the LAD, according to the REGENT study protocol [8]. Baseline coronary angiography and intravascular ultrasound interrogation of LAD distal to stent was performed immediately prior to administration of stem cells. Imaging was repeated at 12 months. Off-line QCA analysis of LAD distal to stent was performed consistent with a typical core laboratory protocol including determination of minimal lumen diameter, MLD, and diameter stenosis, DS). ACOM.PC software (Siemens) was used; image analysis was performed by agreement of two experienced analysts. Cross-sectional plaque images were acquired with 20-MHz phased-array piezoelectric intravascular ultrasound (IVUS, 20MHz Volcano-Philips transducers, gray-scale/VH and ChromaFlo image acquisition), consistent with general requirements and definitions in IVUS and VH-IVUS imaging [17–20]. The transducer pullback speed was 0.5 mm/s (rather than 1.0 mm/s) to minimize the risk of missing the fundamental plaque morphology spot(s) [21]. Radiofrequency backscatter data were collected simultaneously as triggered by the R-wave peak of the patient’s electrocardiogram. The region of interest was defined as LAD distal to the stent(s) implanted during pPCI.
Image analysis was performed off-line. Conventional IVUS parameters, such as minimal lumen area (MLA), plaque burden (PB), and area stenosis (AS) were calculated using QIvus 2.0 software (Medis Medical Imaging Systems, the Netherlands). Corresponding images of baseline and follow-up IVUS examinations were identified by the distance from fiduciary landmarks such as side branches and stent edge. Lesion definition on IVUS required 3 consecutive frames with plaque burden > 40%.
Anonymised, matched angiogram and IVUS runs were analyzed in a random order, with the analysts blinded as to whether a particular dataset included baseline vs. follow-up data.
Besides conventional VH-IVUS measurements [22, 23], qVH analysis was performed using QIvus 2.0 software (Medis Medical Imaging Systems, the Netherlands) customized to enable measurements of several novel quantitative parameters relevant to plaque biomechanics [24, 25] (Figure 1). Matched-image analysis (including ChromaFlo images) was performed to improve the plaque luminal border determination and ensure same-site comparisons at follow-up (Figure 2). This approach overcomes one fundamental image processing limitation of conventional gray-scale and VH IVUS analysis [26]; ie., presence, on a single image, of either colorized flow definition (ChromaFlo mode) or of the VH plaque components (gray-scale and VH-IVUS mode) [21]. Conventional VH (“automatic”, Figure 3) parameters (ie., the total content of necrotic core, NC; fibrofatty component, FF; fibrotic component, FT; and dense calcium, Ca) were analyzed along with the novel qVH measurements such as the minimal fibrous cap (FC) thickness, peak confluent necrotic core (cNC) parameters relevant for plaque biophysics (area, arc/angle, thickness) and other parameters (Figure 3) [21].
Figure 1
Schematic presentation of key components of the atherosclerotic plaque in relation to their architecture and plaque rupture risk [24, 25]. Today, these components can be quantified in vivo with virtual histology (VH-IVUS) using a recently validated novel VH-IVUS algorithm – quantitative virtual histology, qVH [21]. Color-coding is consistent with the VH-IVUS tissue map representation (fibrous tissue – green, necrotic core – red, calcium – white, fibro-fatty – yellow). A – fibrous cap thickness, B – necrotic core area and thickness, C – necrotic core angle. For a typical example of measurements performed in this study see Figure 3
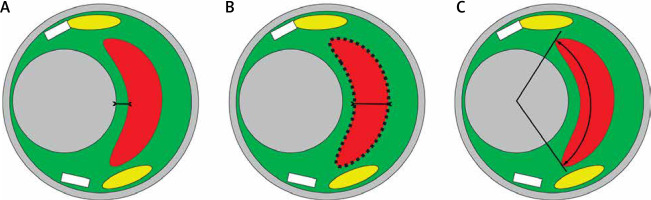
Figure 2
An illustrative example of fundamental importance of performing a matched-image (gray-scale IVUS/VH, A, and ChromaFlo, B) combined analysis to improve accuracy of the plaque luminal border delineation and ensuring same-spot analysis at baseline at follow-up. As the plaque luminal border may not be easy to determine (example in A), matched-image analysis, we introduced a routine application of a “matched image” analysis within the qVH algorithm [21]. This enables minimization of errors in plaque content quantification by VH analysis, that is critical for quantitative evaluation of the plaque composition, and in particular for the qVH measurement of fibrous cap thickness, cf. Figures 1 and 3). “Matched image” analysis plays also an important role in a longitudinal study of plaque evolution that requires a plaque image capture in the same spot (note side branch, SB, as a landmark, to minimize the likelihood of erroneous detection of a change in plaque characteristics as a result of a change in the sampling site along the arterial lumen) [28]
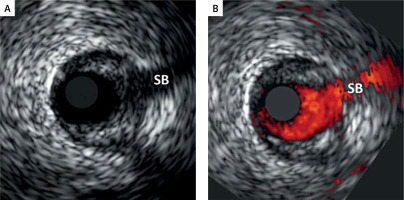
Figure 3
Typical example of quantification of key morphologic parameters of the atherosclerotic plaque using a novel image analysis algorithm (quantitative Virtual Histology, qVH). Commercially available software (QIvus 2.0, Medis Medical Imaging Systems, the Netherlands) was customized for qVH caliper and line tracking and was recently validated [21]. Confluent necrotic core is tracked on the left; numeric results of the measurements are shown in the top panel on the right. Necrotic core area – 1.177 mm2, necrotic core angle – 88.6°, peak necrotic core thickness – 0.536 mm. This contracts with the typical “automatic” software measurements (bottom panel on the right) that consider all pixels in a given color. Note that true necrotic core area is 1.18 mm2 rather than 1.47 mm2. The fibrous cap minimal thickness is 0.31 mm, consistent with a thick-cap fibroatheroma. Also note that the “automatic” software quantification provides a total (per cross-sectional area) content of each of the four principal VH-IVUS–detectable plaque components (fibrous, fibro-fatty, necrotic core, dense calcium), expressed in absolute units, mm2, or as proportion of the plaque area (%) but not of the parameters that are critical for plaque behavior in the context of rupture risk (cf., Figure 1). It may be important to realize that that the “automatic” quantification suffers from several types of artefacts, including the “calcium-associated” (pseudo) necrotic core [21, 29, 30]
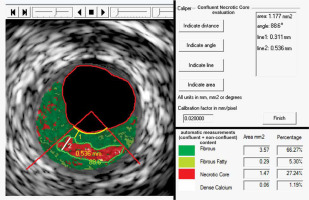
The “peak content” VH frame selection was performed similar to the slice selection on conventional histology by pathologists [27].
Fibroatheroma (FA) was defined as > 10% confluent necrotic core (NC) on three consecutive frames; thin cap fibroatheroma (TCFA) as FA with confluent NC on three consecutive frames and arc of NC in contact with the lumen for 36° along lumen circumference; calcified fibroatheroma (CaFA) as FA with dense calcium (Ca); fibrocalcific plaque (FiCa) as > 10% confluent Ca with no confluent NC; fibrotic plaque (FI) as < 15% fibro-fatty (FF) component and no confluent NC or Ca; pathological intimal thickening (PIT) as ≥ 600 μm thickness for > 20% of the circumference with FF > 15% and absence confluent NC or Ca [18, 20, 22, 23].
The REGENT study and IVUS substudy protocol were approved by the Institutional Ethics Committee. All subjects provided an informed written consent to participate.
Statistical analysis
Statistical analyses were performed using Statistica (version 10.0, StatSoft, Inc., Tulsa, OK, USA) or R (version 2.15.2, R Foundation, Vienna, Austria) software. Categorical data were provided as a number and (if applicable) proportion. Continuous measurements are presented as median values with lower and upper quartiles (Q1, Q3) for all variables as data distribution was mostly non-normal. IVUS imaging numeric data on IVUS examination were expressed as absolute (distance, angle, area) and relative (per cross-sectional plaque area (CSA), proportionate content) values. Differences between the two time points (B/L vs. F/U) were tested using the non-parametric Wilcoxon test. Statistical significance was defined by two-sided p < 0.05.
Results
22 consecutive patients (4 women) aged 59 (52–66) years, enrolled in our center in the REGENT Trial, formed the study group. The patients had been diagnosed with a large ST-elevation anterior wall myocardial infarction and had been treated with primary percutaneous coronary angioplasty of the proximal LAD with thrombus extraction (if feasible) [31, 32] and stent implantation. Peak CK-MB was 460 (345–767) U/l, consistent with a large myocardial tissue loss. On a (median) day 6 (baseline) left ventricular ejection fraction (LVEF) by cMRI was 38.5 (30–41)% and infarct size was 32 (22.2–40.6)%. Stent diameter was 3.0 (3.0–3.5) mm, stent(s) length was 18 (18–26) mm.
On a median day 7 post primary PCI, following a bone marrow aspiration and progenitor cell isolation, a transcoronary infusion of 2.21 (1.63–2.54) × 106 CD34+CXCR4+ autologous cells was performed using a transcoronary cell delivery-dedicated perfusion catheter [11].
Detailed demographic, clinical, and laboratory characteristics of the study group are presented in Table I.
Table I
Characteristics of the study group and medical therapy at discharge
[i] Data are shown as number (proportion, %), or median (Q1–Q3). Total patient number is 22. LVEF – left ventricular ejection fraction, cMRI – cardiac magnetic resonance imaging, ECHO – echocardiography, ACEI – angiotensin-converting enzyme inhibitor, ARB – angiotensin receptor blocker, ADP – adenosine diphosphate; n – number. Troponin I upper limit normal is < 0.1ng/ml; CK-MB upper limit normal is < 24 U/l. See text for details.
At baseline, coronary imaging performed immediately prior to cell delivery showed absence of IRA atherosclerosis distal to the stent by angiography or IVUS in 18.2% patients.
In those patients no new lesions were present when imaging was repeated at 12 months. In the remaining 18 patients, there were 28 stenotic lesions by QCA whereas IVUS revealed 32 lesions. Comparison of baseline vs. 12 month follow-up QCA data showed a numeric increase in the minimal lumen diameter, from 1.43 (1.32–1.78) to 1.7 (1.36–1.94) mm but this increase did not reach statistical significance (p = 0.349). However, there was a statistically significant reduction in diameter stenosis, from 29.5 (25.5–35.5) to 26.5 (21.0–31.5)% (p = 0.012). This is consistent with not only lack of progression but, even more importantly, with angiographic regression of atherosclerosis (Table II).
Table II
Characteristics of angiographic luminal stenoses in the IRA
Parameter | B/L | 12-month F/U | P-value |
---|---|---|---|
MLD [mm] | 1.43 (1.32–1.78) | 1.7 (1.36–1.94) | 0.349 |
DS (%) | 29.5 (25.5–35.5) | 26.5 (21–31.5) | 0.012 |
RD [mm] | 2.11 (1.85–2.55) | 2.2 (1.89–2.6) | 0.525 |
Gray-scale IVUS data are provided in Table III. In brief, the minimal lumen area was 3.19 (2.46–3.92) mm2 at baseline and 3.22 (2.59–3.94) mm2 at 12-months (note a numeric, statistically non-significant increase, p = 0.135). However, there was a statistically significant reduction in IVUS area stenosis, from 33.75 (27.5–39.5) to 31.0 (24.15–38.0)%, p = 0.004. Similarly, IVUS plaque burden decreased from 66.27 (60.22–69.36) to 64.56 (59.93–67.85)%, p = 0.009.
Table III
Gray-scale IVUS characteristics of the study lesions
[i] Data are shown as median (Q1–Q3). Total number of IVUS-detected IRA lesions was 32. IVUS – intravascular ultrasound, MLA – minimal lumen area, PB – plaque burden, AS – area stenosis, DR-LA – distal reference lumen area, PR-LA – proximal reference lumen area, DR-LA – distal reference lumen area, DR-D – distal reference diameter, PR-D – proximal reference diameter. Area stenosis was calculated as 100 – MLA × 100/(0.5 × (DR-LA + PR-LA)).
Quantification of the plaque key components by VH automatic measurements of the “total” content per cross-sectional plaque area revealed a peak necrotic core content reduction from 0.88 (0.45–1.43) to 0.57 (0.34–1.27) mm2 (p = 0.088) while peak fibrotic content increased from 2.5 (1.79–3.1) to 2.82 (1.93–3.66) mm2 (p = 0.018). Total peak fibro-fatty and peak calcific content per plaque area remained stable (p = 0.978 and p = 0.559 respectively), see Figure 4.
Figure 4
Evolution of the key components of atherosclerotic plaques by VH-IVUS. Bars show median values (baseline data in gray, 12-month follow-up data in black). Note a trend to reduction of peak necrotic core component (p = 0.088) and a mild increase in the fibrotic component, consistent with plaque stabilization
NC – necrotic core, FT – fibrotic, FF – fibro-fatty, Ca – dense calcium.
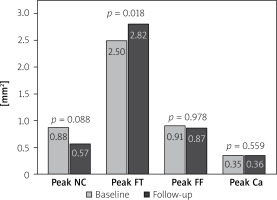
By qVH, there was a numeric reduction in peak confluent necrotic core area from 0.64 (0.2–0.74) to 0.59 (0.31–0.73) mm2 that, however, did not reach statistical significance (p = 0.290). The minimal fibrous cap thickness showed no change, with 0.15 (0.13–0.19) mm at baseline and 0.16 (0.13–0.2) mm at 12 months (p = 0.646). Overall, there was no progression to unstable plaque characteristics by qVH. Full data for conventional (“automatic” measurement of the “total” (fibrotic, fibro-fatty, necrotic core and dense calcium) per cross-sectional plaque area content) and qVH parameters (including other confluent necrotic core measurements and fibrous cap thickness) are provided in Table IV.
Table IV
VH-IVUS characteristics of the study lesions
Variable | Baseline | 12-month F/U | P-value |
---|---|---|---|
Peak total content per plaque CSAa: | |||
Peak NC (%) | 23.5 (16.18–34.37) | 23.45 (12.26–29.05) | 0.151 |
NC MLA [mm2] | 0.37 (0.23–0.82) | 0.36 (0.11–0.68) | 0.487 |
NC MLA (%) | 13.79 (8.27–22.01) | 13.33 (4.57–21.5) | 0.305 |
Peak FT (%) | 70.41 (65.2–75.41) | 75 (68.67–79.79) | 0.004 |
FT MLA [mm2] | 1.9 (1.35–2.48) | 1.89 (1.47–2.81) | 0.230 |
FT MLA (%) | 56.15 (49.15–67.74) | 64.88 (55.83–70.66) | 0.009 |
Peak FF (%) | 24.75 (15.65–41.1) | 23 (16.48–31.48) | 0.708 |
FF MLA [mm2] | 0.51 (0.29–0.86) | 0.51 (0.24–1.07) | 0.805 |
FF MLA (%) | 17.24 (7.9–25.24) | 15.25 (9.13–31.1) | 0.562 |
Peak Ca (%) | 12.93 (7.94–19.8) | 14.7 (7.17–23.4) | 0.797 |
Ca MLA [mm2] | 0.13 (0.02–0.31) | 0.15 (0.05–0.4) | 0.509 |
Ca MLA (%) | 3.7 (0.58–11.33) | 3.91 (1.76–14.1) | 0.207 |
Peak total FF + NC [mm2] | 1.75 (1.08–2.37) | 1.55 (0.94–2.43) | 0.786 |
Peak total FF + NC (%) | 43 (36.7–49.11) | 38.93 (36.1–46.71) | 0.331 |
Confluent contentb: | |||
confl. NC area [mm2] | 0.64 (0.2–0.74) | 0.59 (0.31–0.73) | 0.290 |
confl. NC area per plaque CSA (%) | 12.52 (6.45–20.95) | 11.55 (7.45–19.15) | 0.695 |
confl. NC arc [°] | 64.55 (34–88.55) | 58.1 (44.2–83.49) | 0.937 |
confl. NC thickness [mm] | 0.42 (0.28–0.46) | 0.39 (0.32–0.48) | 0.875 |
confl. Ca area [mm2] | 0.36 (0.23–0.59) | 0.41 (0.25–0.69) | 0.155 |
confl. Ca arc [°] | 53.4 (44.1–71.3) | 61.9 (40.6–73.5) | 0.991 |
Minimum FC thickness [mm]c | 0.15 (0.13–0.19) | 0.16 (0.13–0.2) | 0.646 |
Data are shown as median (Q1–Q3); n = 32. VH – virtual histology, CSA – cross-sectional plaque area (CSA = EEM area – lumen area, EEM – external elastic membrane), MLA – minimal lumen area, NC – necrotic core, FT – fibrotic component, FF – fibro-fatty component, Ca – dense calcium component, confl. – confluent. ‘MLA’ indicates measurements performed at the minimal lumen area spot.
a Conventional VH-IVUS parameters (“automatic”, provided by the routine VH software, expressed as a peak total content per plaque).
b Peak confluent content per plaque (determined using qVH algorithm [21]).
Qualitative analysis of phenotypes of the 32 plaques present at baseline showed the following: pathological intimal thickening (PIT) – 2, fibrotic (FT) – 0, fibrocalcific (FiCa) – 20, “thick”-cap fibroatheroma (FA) – 7, “thick”-cap calcified fibroatheroma (CaFA) – 3, thin-cap fibroatheroma (TCFA) – 0, calcified thin-cap fibroatheroma (CaTCFA) – 0.
At 12 months, 2 (“thick”-cap) fibroatheromas showed transition to (“thick”-cap) calcified fibroatheromas. In addition, 1 (“thick”-cap) fibroatheroma evolved to a fibrocalcific lesion. Other plaques remained stable (low-risk) phenotype (absence of evolution), and no new lesions occurred. Full data for plaque phenotypes at baseline and at 12 months are presented, together with representative examples of the plaque (limited) evolution, in Figure 5.
Figure 5
Evolution of infarct-related artery atherosclerotic plaque phenotypes in this study (A) and typical examples (B, C). Note that the overall plaque phenotype remained stable – both taken as an absence of “unstable” plaque phenotypes (thin-cap fibroathaeroma, calcified thin-cap fibroatheroma) and (also) as a lack of any major changes in the individual plaque phenotypes (A). Two fibroatheromas (ie., “thick”-cap fibroatheroma) progressed to the calcified fibroatheroma phenotype and one other to the fibro-calcific phenotype (both phenotype changes consistent with an increase in plaque stability); the remaining 30 plaques maintained their phenotype (A). B is an example of a stable calcified fibroatheroma; C shows an evolution from a (“thick”-cap) fibroatheroma to a fibro-calcific plaque. Also note the importance of the use of landmarks to ensure that evaluation occurs at the same “site” at baseline and at 12 months
FiCa – fibrocalcific, CaFA – calcified fibroatheroma, FA – fibroatheroma (“thick-cap” fibroatheroma), PIT – pathological intimal thickening.
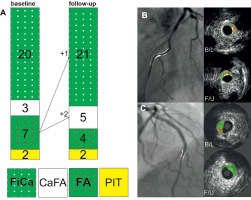
Discussion
To monitor evolution of coronary artery atherosclerosis after using the intracoronary route for stem/progenitor cell delivery, this study used both conventional imaging of the arterial lumen and plaque (quantitative angiography, intravascular ultrasound) and a novel ultrasonographic image analysis algorithm that enables precise quantification of key functional components of the atherosclerotic plaque (quantitative virtual histology).
Our principal finding is absence of atherosclerosis progression in the infarct-related artery 12 months after using it as a route for perfusion administration of stem cells. Moreover, follow-up evaluation with the index techniques of visualization documented a moderate reduction in plaque burden and stenoses severity. The latter might result from maximized pharmacologic therapy in the study patients and/or it could be mediated by stem cell-dependent mechanisms.
Some initial studies labelled progression of IRA atherosclerosis after stem cell transcoronary administration as a stem cell-mediated mechanistic adverse effect [15, 16]. If confirmed, this would pose an important limitation in applicability of the transcoronary route for delivering cell-based therapies. This is important because the transcoronary route plays a fundamental role in administration of cell-based therapies to compromised myocardium. In particular, as demonstrated by labelled cell myocardial uptake studies [11, 12, 33], this route is mostly effective (with its efficacy degree related to the delivery techniques and cell types). Easiness to use and a good safety-and-feasibility to efficacy balance are important clinical advantages.
Vanderheyden et al. [15] and Mansour et al. [16] suggested that CD133+ (endothelial progenitor)–enriched bone marrow stem cells might stimulate in-stent neointimal proliferation and progression of distal-to-stent atherosclerosis. Such a potential effect, understood as a “tradeoff” inherent to therapies designed to enhance cardiac repair [16, 34], raised concerns with regard to using the transcoronary route for progenitor/stem cell delivery in further clinical trials. Some bench and animal model data seemed to support the “tradeoff” concept. For instance, a study of hindlimb ischemia in apolipoprotein E-knockout mice by Silvestre et al. [35] reported that transplantation of 1 × 106 bone marrow-derived mononuclear cells from wild-type animals accelerated atherosclerosis by inducing a significant (by ≈ 50–70% ) increase in atherosclerotic lesion size in absence of an effect on plaque composition. At least one other study in the apolipoprotein E-knockout murine model suggested that transfer of bone marrow cells and endothelial progenitor cells may result in promotion of atherosclerosis [36]. George et al. [36] associated bone marrow and endothelial progenitor cells with an increase in the atherosclerotic lesion size (via increasing lipid cores) whereas endothelial progenitor cells were also claimed to enhance thinning of the fibrous cap and thus reduce plaque stability [36].
Although bone marrow cells can differentiate into vascular cells that participate in the pathogenesis of atherosclerosis [37], other progenitor cell types – such as Wharton Jelly mesenchymal stem cells (umbilical cord stem cells) – have been found to stimulate neovascularization (and thus improve oxygen supply to the ischaemic tissue) in absence of any detectable stimulation of atherosclerosis progression [38]. These different (and seemingly contradictory) findings suggest that a “pro-“ vs. “anti-“ atherosclerotic (or neutral) effect of stem/progenitor cells may depend not only on the cell type but also, to some extent, on the study model, making detailed human studies (like ours) particularly relevant. Several recent reports indicate that mesenchymal stem cell transplantation may alleviate atherosclerosis by promoting an anti-inflammatory environment and inhibiting the atherosclerotic pathways [39, 40].
CD34+CXCR4+ stem/progenitor cells are spontaneously mobilized in AMI [41], and the magnitude of mobilization shows a positive correlation with improvement in myocardial contractility at 12 months [41]. This is in agreement with the finding that higher counts of circulating progenitor cells, including CD34+CXCR4+ cells, are associated with better vascular function in the presence of risk factors [42]. In a recent study in 1366 subjects enrolled into the Emory Cardiovascular Biobank, lower counts (≤ median) of CD34+/CXCR4+ and CD34+/VEGFR2+ cells independently predicted all-cause mortality (HR = 1.46, 95% CI: 1.06–2.01, p = 0.02 and HR = 1.59, 95% CI: 1.15–2.18, p = 0.004) and cardiovascular death/myocardial infarction (HR = 1.50, 95% CI: 1.04–2.17, p = 0.03 and HR = 1.47, 95% CI: 1.01–2.03, p = 0.04) over a period of 3 years, compared to the individuals with lineages above the median value [43]. These findings are consistent with the anti-atherosclerotic and plaque-stabilizing effect of CD34+CXCR4+ cells suggested by our present findings (Figures 4, 5, Tables II–IV).
Recent work by the teams of Assmus et al. [9] and Qiu et al. [44] provides some evidence against any relevant pro-athrosclerotic effect of transcoronary-administered stem/progenitor cells in patients with AMI. Retrospective QCA measurements of the infarct target vessel in 83 patients with AMI treated with stent-assisted PCI (a control group) and in 83 patients receiving additional intracoronary progenitor cell infusion at 5 days post pPCI, with coronary angiography repeated at 4 months, showed that the late loss as a measure of neointima formation was similar between the control and the cell-treated group at follow-up (0.9 ±0.8 vs. 0.9 ±0.7 mm, p = 0.9) [9]. Stent restenosis rate was also comparable in both groups (35% control vs. 27% cell-treated group, p = 0.2), consistent with lack of any stimulatory effect of stem/progenitor cells on in-stent proliferation. The latter would have been, in any case, highly unlikely as the cells are typically administered distal to the stent. Unfortunately, any detailed assessment of atherosclerosis distal to the IRA stent was lacking [9]. Rather, these investigators based their conclusion only on a relatively low (28.9%) target vessel revascularization rate during a median of > 3 years of follow-up [9]. More detailed work by Qiu et al. [44] compared baseline and 4-month follow-up angiograms and IVUS images from STEMI patients treated with CD 133+ stem cell intracoronary injection after pPCI (n = 17) versus those obtained in patients who received placebo (n = 20). IRA analysis revealed similar changes in distal nonstented segment plaque burden between the stem cell and placebo groups (B/L – 41.9 vs. 37.4%, p = 0.56; F/U – 46.7 vs. 40.9%, p = 0.17) [44]. Findings also were similar for the distal non-culprit segment in patients receiving vs. not receiving stem cells [44]. Furthermore, in the contralateral coronary artery, there were no differences in changes in MLA, plaque burden, or attenuated plaque between stem cell and placebo patients [44]. The authors concluded that intracoronary injection of CD133+ bone marrow stem cells had no IVUS-detectable effect on neointimal hyperplasia or atherosclerosis progression in either IRA or contralateral arteries [44]. In aggregate, these studies [9, 44] taken together with our present results (Tables II–IV) refute early data by Vanderheyden et al. [15] and Mansour et al. [16]. Moreover, our findings indicate regression of IRA atherosclerosis (Tables II–IV) in patients subjected to delivery of progenitor/stem cells via the IRA.
Intravascular imaging plays a fundamental role in longitudinal studies of atherosclerosis evolution [45–50], including evaluation of plaque morphology (and its changes) in relation to atherosclerosis-mediated cardiac adverse clinical events such as MI and cardiac death [48, 51–54]. Intracoronary imaging is particularly important for its increased accuracy (against traditional planar analysis of intraarterial angiography images) of lesion detection and evaluation of its severity and morphology. Indeed, 87.5% of IVUS-identified lesions in this study could be detected with QCA (see Table III vs. Table II). Importantly, our study applied not only a range of routinely available state-of-the-art techniques (such as conventional IVUS, “automatic” VH image analysis limited to quantification of the “total” per plaque cross-sectional area NC/FF/FT/Ca and prone to several fundamental analysis artefacts [29, 30, 55] and other constraints [56]) but also a novel algorithm of qVH evaluation [21]. Recently validated qVH algorithm [21], with its precise evaluation of fundamental plaque biophysics-based characteristics [23, 24] (Figure 1), has been presently introduced for the first time in a longitudinal clinical study use (Figures 2, 3, Table IV). Because of significant limitations of conventional VH-IVUS image quantification revealed in prior studies, qVH appears to have several fundamental advantages [21] that may improve IVUS-based longitudinal research of coronary atherosclerosis.
Recent analysis by the Mayo Clinic group applied conventional VH-IVUS to evaluate plaque evolution in a series of 80 heart transplant receipients [57]. The study, using “automatic” (total content per plaque area) quantification of the necrotic core found that patients with greater yearly median change in necrotic core had lower levels of both CD34+CD133+ circulating progenitor cells (225 (130–518) vs. 470 (285–760) per 100 000 counts, p = 0.006) as well as CD34+ cells (860 (485–2223) vs. 1840 (910–2590) per 100 000 counts, p = 0.05) [54]. Also, patients with TCFA at baseline IVUS examination had significantly lower levels of circulating CD34+ CD133+ cells (p = 0.03) [54]. These findings are consistent with a reduced progression of cardiac allograft vasculopathy and a reduction in cardiovascular events in relation to higher levels of circulating CD34+CD133+ progenitor cells [54].
Both IVUS-detected TCFA and TCFA by optical coherence tomography (OCT) is typically located proximal to the maximal lumen stenosis (minimal lumen area site), consistent with a “fire-and-smoke” mechanism [22]. The “fire-and-smoke” mechanism, initially identified for its pivotal role in pathology of acute coronary syndromes [58, 59] is also relevant as a basis for an increased risk of major adverse cardiac events in clinically stable patients with TCFA [53]. Although VH-IVUS detection of the atherosclerotic plaque 4 fundamental components has been validated against ex-vivo histology [60–62], the discriminatory power of conventional VH-IVUS findings (particularly in relation to future cardiac events) may be limited by use of a “total” (pixel-colour-generated) proportional content of FF/FT/NC/DC. This is now changed with plaque biophysics-based qVH evaluation (Figures 1, 3, Table IV) that we recently validated [21]. Further limitations of the conventional VH-IVUS analyses arise from inability to quantitatively discriminate artefacts [29, 30], use of different TCFA definitions use across studies [22, 48, 56, 63] as well as the “requirement” of the TCFA phenotype “presence” in 3 consecutive VH-IVUS frames” to determine the lesion as TCFA [23]. Because the radiofrequency image capture is triggered by the R have on ECG [22], the latter results in the dependence of TCFA identification on heart rate at the time of IVUS interrogation which is clearly flawed [55]. These limitations of conventional analysis lead to fragility of TCFA identification in over 40% coronary plaques getting labelled as a “TCFA” [56]. Those, very significant, considerations, indicate an important role for the recently validated qVH algorithm [21] that exhibits a very high reproducibility and minimal inter-observer and inter-transducer variability [21]. These features, suboptimal with conventional VH-IVUS image analysis algorithms [64] are critical in longitudinal studies of atherosclerosis.
One important advantage of IVUS, a sound-based modality, is its tissue penetration of ≈10 mm for a 20 MHz transducer [65]. This enables, in most cases, visualization of the entire coronary atherosclerotic plaque thickness that is critical for determining architecture of its key components [24, 25, 65] (cf., Figure 3) and for predicting plaque behaviour including the risk of rupture [24, 25, 66]. Axial resolution of 20M Hz IVUS catheters (such as the ones used in this study) is ≈120 µm and lateral resolution is ≈ 200 µm [65]. OCT is a light-based imaging modality whose resolution (12–15 µm axial, 20–40 µm lateral) is superior to that of IVUS [65]. This, however, occurs at the cost of very limited tissue penetration (≈1.0–2.5 mm) that enables to capture with OCT only the para-luminal segment of the atherosclerotic plaque [65]. Apart from the OCT inability to fully quantify critical constituents of the plaque (Figure 1), OCT is prone to image artefacts that may result (with histology as the benchmark) in misclassification of intimal thickening as fibroatheroma or thin cap fibroatheroma [67]. Adding VH-IVUS to OCT significantly reduced the error rate, indicating an important role for combined (“hybrid”) IVUS-OCT imaging, taking advantage of the much greater tissue penetration with IVUS [65, 66]. However, it needs to be recognized that plaque progression and rupture are not solely determined by plaque configuration but also by mechanical factors [24, 25] that may induce instability and by active inflammation [21, 66].
One fundamental limitation of this study is the sample size that, by some, may be considered moderate. Another is lack of cohort not receiving cell administration. While absence of a control group of IRA-imaged patients not subjected to stem cell administration does not significantly affect conclusions from this study with regard to absence of atherosclerosis progression in association with transcoronary stem cell administration, it affects any insights into the mechanism(s) of atherosclerosis regression that we have observed in cell-treated arteries. In particular, regression of atherosclerosis may occur in relation to intensive medical therapy [45–47]. We received no approval to repeat invasive imaging in in the control group patients. Other limitations include our lack of evaluation of non-IRA arteries. However, any stem cell-mediated effects on non-IRA coronary arteries are not only unlikely with trans-IRA cell delivery [44], and those would be expected to occur similarly in arteries other than the coronaries. Axial resolution of IVUS (≈120 µm in 20-MHz transducers) is rather poor in relation to OCT [65]. Thus IVUS is unable to measure precisely the thickness of coronary artery rupture-prone fibrous cap whose histologic thickness is determined at ≈65 µm [27]. Of note, this is not a limitation in the carotid arteries where the fibrous cap critical for rupture risk is ≈200 µm [21]. Importantly, this (general) IVUS limitation is not directly applicable to data from this study because of absence of TCFA(s) amongst lesion phenotypes (Figure 5). As we identified a consistent (accross the imaging techniques) and statistically significant reduction in atherosclerosis over 12 months after transcoronary stem cell delivery, it is unlikely that a larger sample size would yield qualitatively different results. Nevertheless, it remains to be evaluated whether (and to what extent) the present findings are applicable to other stem cell types and doses.
Conclusions
This study, using both classic and novel imaging techniques including quantitative virtual histology, shows lack of any stimulatory effect of transcoronary stem cell transfer on coronary atherosclerosis. 12 months after transcoronary cell administration there was a reduction in stenosis severity by both angiography and intravascular ultrasound while virtual histology and quantitative virtual histology indicated stable – and further stabilizing – plaque phenotypes. This is reassuring for continued use of the transcoronary route for delivery of therapeutic cells to compromised myocardium. Further work is required to determine whether, and to what extent, the moderate reduction in plaque burden and atherosclerotic lumen reduction in mid-term after stem cell delivery results from optimized pharmacotherapy and/or transcoronary stem cell transfer.