INTRODUCTION
The classification of extracellular vesicles (EVs) – structures released by cells that have been observed since the 1960s, carrying various types of chemical compounds as their contents – requires looking at them in terms of their size or functionality. This first parameter allows us to distinguish small vesicles: exosomes (30-150 nm) and ectosomes (100-1000 nm) and large vesicles, including: migrasomes (500-3000 nm) [1], apoptotic bodies (1000-5,000 nm) [2] and oncosomes (1000-10,000 nm) [3]. Another parameter – functionality – refers, among other things, to the content of the vesicles, the type of cells from which they derived or the target cells.
Extracellular membrane vesicles were initially assigned by researchers the primary role of getting rid of unnecessary cellular contents and removing unnecessary or harmful metabolites. During studies of platelets in the 1960s, observed structures that were later classified as ectosomes were called “platelet dust” [4]. Exosomes, slightly smaller vesicles, were first noticed during studies of blood cells in the 1980s, and it was shown that during the process of reticulocyte maturation, vesicles containing the transferrin receptor are secreted outside the cell [5, 6]. Gradually, research showed that the content of exosomes is also crucial in communication between cells and can be treated as a physiological determinant of the normal or pathological condition of the body [7]. The molecular cargo carried in the extracellular space and over longer distances is found in various body fluids: blood, saliva, urine, sperm; peritoneal, amniotic and cerebrospinal fluid carry important information, sometimes influencing the functioning of distant cells [8, 9]. The content of EVs depends largely on the cell type origin but based on research it has been possible to determine that in general their cargo consists of nucleic acids (DNA, RNA, miRNA) as well as proteins and lipids and, in the case of apoptotic bodies, also the organelles of a cell that has undergone apoptosis. Initially, the term secretome was used only for substances secreted directly into the extracellular space [10]. Currently, this concept has also been supplemented with the content found in EVs. The concept of secretome may refer to any body fluid in the examined organism, but also to the content of the culture medium into which cultured cells release organic substances and vesicles with their cargo [11].
Exosomes are the most thoroughly studied EVs and the most often considered for therapeutic applications. As one of several elements constituting the population of vesicles, i.e. structures differing in their physical and biochemical properties as well as their sources of origin, they were first described as structures released with the participation of the cell membrane into the extracellular space during the maturation of reticulocytes, and are currently classified as EVs with a diameter of 50-150 nm of endosomal origin. Exosomes isolated from body fluids are characterized by significant differences in size, structure and content, which is of course important for understanding their functions. One of these functions is, for example, modulation of the immune response of cells involved in the body’s response to cancer. In addition to exosomes, it is particularly influenced by other microvesicles called oncosomes, released by cancer cells and promoting angiogenesis and metastasis [12, 13]. Within the nervous system, the communicative and regulatory activity of exosomes affects the maintenance of homeostasis, ensuring the proper functioning of the central nervous system (CNS), affects neurogenesis, synaptic activity and plasticity, neuronal myelination, and has a protective and regenerative effect after potential damage or disease (Figure I).
Figure I
Classification of extracellular vesicles considering their size and origin with the markers of membrane characteristics
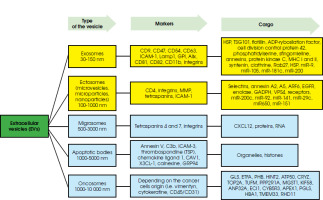
The transport of various substances enclosed in vesicles also seems to be very promising when it comes to therapeutic prospects in numerous diseases, and the possibility of their crossing the blood-brain barrier (BBB) particularly indicates diseases of the CNS. Due to the overlapping size of specific types of vesicles and the difficulty in clearly defining markers specific to each type, as well as the difficulty of reliably isolating a specific population of vesicles, the International Society for Extracellular Vesicles (ISEV) recommends using the general term ‘extracellular vesicles’ – EVs [14].
On the other hand, due to the predominance of studies described as those concerning exosomes over other vesicle populations, further information contained in this work will primarily concern these types of EVs.
METHODS
This review was performed following an electronic search of the database PubMed/Medline and Web of Science for English-language articles between 2010 and 2024 in the fields of medicine, molecular biology, and biochemistry. Keywords searches included combinations of the following terms: “extracellular vesicles” OR “exosomes” AND “neurodeg*” AND “microRNA” OR “miRNA” AND “AD” OR “PD” OR “ALS” OR “HD”. Articles had to be original work or reviews.
THE BIOGENESIS AND STRUCTURE OF EXOSOMES
The biogenesis of EVs occurs with the participation of the cellular membrane, but different mechanisms are involved. Ectosomes, also called membrane particles, are formed by the budding of vesicles directly from the cell membrane. In a similar way, the larger vesicles called oncosomes and apoptotic bodies are formed [15]. In the form of elongated, tubular structures, with the final extension containing cytoplasmic granules, vesicles called migrasomes are also developed via membrane budding [16].
Initially, exosomes are formed by the inward budding (internalization) of structures from the cell membrane called intraluminar vesicles, forming early endosomes, and then transforming into multivesicular bodies (MVBs). The first step is the inward curvature of the cell membrane, which is facilitated by multisubunit proteins such as calveolins and clathrins [17, 18]. The composition of the membrane of the inner vesicle – the future exosome – is slightly different from the parent cell membrane, being more rigid due to the incorporation of phosphatidylserine into it and an increase in cholesterol content. The MVB contains numerous vesicles that are actual exosomes. They come from numerous invaginations of the endosome membrane into its lumen. MVBs can be degraded intracellularly by lysosomes, but they can also be redirected toward the cell membrane, which probably depends on the type of cargo transferred. The next stage is MVB transport, which involves the ESCRT (endosomal sorting complex required for transport) protein complex and dynamins. Proteins of this complex also influence the distribution and organization of exosomes inside MBV. The existence of another route of MVB transport involving tetraspanins, which are also markers of exosomes and membrane lipids, cannot be ruled out [19]. In order to release the contents, MVBs fuse with the cell membrane by exocytosis. The secretion of vesicles outside the cell requires additional processes. In the first stage, MVBs dock at the inner side of the cell membrane and fuse with it, secreting exosomes and other vesicles. The mechanism of this secretion is usually dependent on calcium ions, although a calcium-independent fusion involving the soluble N-ethylmaleimide-sensitive factor-activating protein receptor (SNARE) has also been detected [20]. Remodeling of the cytoskeleton, recycling of endosomes with the participation of the Rab11 protein, as well as the participation of the lipid metabolism pathway, including cholesterol, ensuring appropriate membrane fluidity, is extremely important in the process of secretion [21].
While still inside the cell, MVBs interact with the membranes of the endoplasmic reticulum (ER). If the sites of contact between MBV and the ER are damaged, uncollected cargo may accumulate in the ER and be degraded via the proteasomal pathway. If this pathway is dysfunctional, e.g. in neurons, an excessive accumulation of misfolded proteins may occur. Then, the MVBs release their contents, i.e. exosomal vesicles, into the extracellular space [22]. A diagram of the formation of different types of vesicles is shown in Figure II.
Figure II
Biogenesis, secretion and schematic structure of extracellular vesicles (created in BioRender.com)
MVBs – multivesicular bodies; endocytosis – the process of the invagination of a cell membrane into the cell body producing vesicles; exocytosis – a process of fusing vesicles with the plasma membrane and releasing the cargo to the extracellular space; budding – the process of the envagination of the cell membrane and external vesicle formation; blebbing – protrusion of the apoptotic cell membrane leading to bulge formation
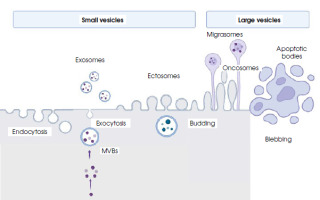
Due to the complexity of exosome biogenesis and transport, research on these processes constantly reveals new elements, especially the role of proteins involved in various stages of exosome formation. Some of them accompany exosomes until they are released from the cell. These include: calveolin and clathrin, dynamin, neutral sphingomyelinase, small GTPases (RaIA/RaIB, Rab31) and others. The components of the ESCRT complex also participate in the maturation of these exosomes: CHMP 4B/7, ALIX, TSG101, GPR143 [23, 24]. Moreover, the cargo sorting carried by exosomes involves – among other proteins like VAP, exocyst complex, V-ATPase, LAMP2A, LAP4M4B, LC3, IST – proteins from the Eps15 Homology Domain (EHD) family, CD2AP and, additionally, proteins from the actin family together with other proteins are involved in the secretion of exosomes outside the cell [25].
METHODS OF ISOLATION OF EXTRACELLULAR VESICLES
To separate EVs, isolation methods should be used to extract the appropriate fraction with the highest possible efficiency due to their small size. Examples of approaches to isolation methods are presented in Table 1. Individual methods are often combined together to obtain the best possible effectiveness [24, 26]. The choice of method also depends on whether we are dealing directly with a biological sample originating, e.g., from body fluids, or whether it is a sample from the culture medium in which the cells were located. The choice of the isolation method should be carefully considered before starting the analysis and should also take into account what amounts/volumes of exosomes will be needed and what contaminants generated during the isolation process should be avoided. The development of new exosome isolation techniques, especially those based on microfluidic technology, is described in the work of Chen et al. [27]. A comparison of the commercial isolation kits was described in the study of Ding et al. [38].
Table 1
Methods for the extracellular isolation of vesicles
Method | Principle | Advantage | Disadvantage | Ref. |
---|---|---|---|---|
Ultra-centrifugation | Separation of particular elements according to size and density in the centrifugal force | A relatively cheap, large-volume sample may be processed, only centrifuge is needed | Time-consuming, low yield of exosomes, may cause damage | [28, 29] |
Density gradient ultra-centrifugation | Separation of particular elements according to size and density in the centrifugal force and in the density gradient | Effective removal of elements not related to exosomes; gradient can be used to adjust the resolution of the separation | No distinction between exosomes of similar size but different origin/structure | [30] |
Precipitation using PEG/other substances | PEG accelerates the precipitation of small particles (viruses, exosomes) during centrifugation | Easy to carry out, does not require specialized equipment, can also be used with large sample volumes | Relatively high reagent costs; co-precipitation with protein aggregates and other compounds | [31] |
Size exclusion chromatography | Size-based separation | Relatively easy to carry out, preserves the vesicles’ integrity | Time-consuming, possible contamination with other particles | [32] |
Magnetic beads technique | Antibodies coated on the beads selectively capture the antigen in the vesicle membrane | High specificity, easily commercially available | Not suitable for large volume samples | [33] |
Microfluidic technique | Capturing structures of appropriate size on nanomembranes and nanowires | Fast, high-throughput, cost-effective, enables simultaneous characterization of isolated exosomes, small sample volumes | Requires experience, standardization and validation recommended when examining larger cohorts, hard-to-access technology | [34-36] |
Commercial isolation kits | Various technologies, e.g. precipitation with resin | Fast, centrifugation is not always necessary | Not suitable for large volume samples | [37] |
The importance of choosing the method of isolation and further preparation is important because the standardization of exosome tests is strongly recommended by the ISEV, both in terms of their acquisition and characterization. The so-called minimum requirements are presented and updated by ISEV. The last such update took place in 2022/23 [39].
The identification of the origin of vesicles within the collected material is based on the determination of the protein markers on their surface. Knowing that the membrane of the vesicles is the membrane of the source cell releasing this particular vesicle, the detection is made by precipitation with antibody-based immunoaffinity capture or direct antibody immunoaffinity isolation methods. From collected plasma different types of brain-derived EVs may be recognized: neuron-derived EVs (NDEs) with CD171+/CD19+, L1CAM, SNAP25 staining; astrocyte-derived EVs (ADEs) with EAAT1+/CD9+ or GLAST AQP4/GFAP staining and oligodendrocyte EVs with MOG+/CD9+ and CNP/OMG staining [23, 40].
THE IMPORTANCE OF TRANSPORTING THE EXOSOMES’ CARGO WITHIN THE BODY
Exosomes that have been released into the extracellular space travel with body fluids over longer distances and, after being absorbed into the surface of target cells, are internalized and release their contents into the cell [41]. This process plays a huge role in communication between cells and is essential as a physiological element of cellular interference. Such communication, though in a pathological mechanism, also occurs when both normal and, above all, cancer cells release EVs (e.g. oncosomes), transporting their cargo to adjacent and distant cells, significantly influencing their proliferation, migration, invasiveness or adhesion and resistance of target cells to anticancer drugs [42]. Bioactive substances contained in EVs also influence the processes of angiogenesis, antigen presentation, immune response, cell apoptosis and intracellular signal transduction. Most information about the importance of exosomes and other vesicles comes from research on immunological processes, including the response to the presence of cancer cells [43].
The attachment of vesicles to target cells is related to the presence of integrins on the surface of EVs, which are transmembrane proteins called glycoproteins. The high variability in their structure, additionally provided by alternative splicing, determines their affinity and binding to various ligands. Known ligands for integrins are fibronectin, vitronectin, collagen and laminins, and their combination may induce signal transmission to the cell nucleus [44]. From a physical point of view, integrins facilitate the anchoring of the exosome to the target cell membrane and its further integration into the cell. The type of integrin on the exosome surface determines, to some extent, their purpose. For example, it was noticed that exosomes derived from T lymphocytes and having α4β7 integrin on their surface preferentially combine with intestinal endothelial cells infected with the hepatitis E virus [45] and, as Hoshino et al. [46] reported, cancer exosomes that display integrin α6β4 were preferentially taken up by the lung, whereas those displaying αVβ5 were taken up by the liver. This shows that vesicles with surface expressions of α6β4 and α6β1, by combining with fibroblasts and epithelial cells, regulate the formation of metastases in the lung. This also means that metastasis can be limited by inhibiting the expression of specific integrins on vesicle membranes or inhibiting the binding of integrins to target cells. However, not all studies confirm the essential role of integrins in the structure of the membrane of EVs, which means that there is a need for further, intensive research on various types of EVs.
PROTEINS RELATED TO NEURODEGENERATIVE PROCESSES AS PART OF THE CONTENT OF EXTRACELLULAR VESICLES
The most frequently studied degenerative diseases of the CNS in terms of the role and content of EVs are Alzheimer’s (AD) and Parkinson’s (PD). Both diseases are characterized by the presence of misfolded proteins, the presence of which can also be detected outside the nervous system. AD is characterized by the participation of aggregated amyloid β particles in the form of extracellular amyloid deposits [47]. Inside the cells, neurofibrillary tangles composed of excessively phosphorylated tau protein can be observed. In the case of PD, misfolded α-synuclein is found in dopaminergic neurons of the substantia nigra in the brains of patients [48].
In its physiological form α-synuclein regulates the turnover of synaptic vesicles and the release of neurotransmitters, although its direct location within the neuron cell ending is not precisely known. Most likely, it connects with the membrane of the synaptic vesicles and perhaps that is why it influences their endo- and exocytosis and the release of neurotransmitters from synaptic and secretory vesicles through calcium-triggered exocytosis [49, 50]. Pathological α-synuclein is also detected in organs and body fluids other than the CNS, which indicates the important role played by synuclein transport. One of such less known methods is the use of tunneling nanotubes, connecting brain-microvascular endothelial cells and pericytes, which allows the creation of a tunnel passage cross the blood-brain barrier and the entry of alpha-synuclein from the periphery into the brain [51].
According to the prion theory, which refers to AD and PD but also to other neurodegenerative diseases involving misfolded proteins, a protein with incorrect conformation can serve as a matrix for normal protein molecules and propagate the spread of toxic protein fragments to other cells [52]. It seems that EVs play an essential role in the transport of such misfolded proteins, initiating pathological processes in previously normal cells. This theory has been confirmed, among others, in multiple models of PD by demonstrating that newly synthesized α-synuclein in the form of monomers and aggregates is released into the extracellular space [53-55]; moreover, the presence of α-synuclein has been demonstrated in the body fluids (cerebrospinal fluid, serum) of healthy people and patients with PD and the exosomes obtained from the brain tissue of a PD patient and injected into mouse brains caused the α-synuclein contained in them to be taken up by neurons and astrocytes [56]. It was also observed that the α-synuclein present in exosomes from PD patients caused changes leading to the degeneration of dopaminergic neurons, and activation of microglial cells and motor functions in mice [57].
Figure III
Implication of cellular processes for the accumulation of misfolded proteins (created in BioRender.com)
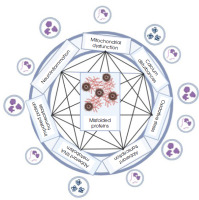
In the case of AD, it is known that the APP protein, which is synthesized in the ER and transported to the Golgi apparatus, is then delivered close to the cell membrane or to early endosomes. Physiologically, in the first case, the protein is cleaved by α-secretase and enters the non-amyloidogenic pathway. However, if APP absorbed into the endosome is cleaved by β-secretase, creating a soluble APPβ fragment and a β-C-terminal fragment, it will be directed towards the pathological processing of this protein. The next stage of APP protein processing carried out by γ-secretase may take place both in the cell membrane and in the endosomal-lysosomal compartment [58]. It has also been observed that inhibition of the ESCRT protein complex, closely related to exosome maturation, contributes to the accumulation of APP protein in endosomes. Such excessively enlarged endosomes likely release more vesicles filled with amyloid beta [59]. Inhibition of the ESCRT complex may also reduce the transport of APP to lysosomes, ultimately causing intracellular Aβ levels to build up. Persistent secretion of EVs containing significant amounts of Aβ was observed in glioma cells with dysfunctional lysosomes and abnormal presenilin due to mutations [60]. But APP processing can also occur extracellularly, as demonstrated by observing the activity of γ-secretase, which processed APP protein in EVs isolated from the brain tissue of a mouse model of AD and incubated in the absence of cells [61].
In models and patients with AD, in which the key signs are the presence of amyloid β, neurofibrillary tangles containing tau protein, and the accumulation of senile plaques along with the loss of neurons and synapses, the content of exosomes derived from neurons can provide a lot of information about the degree of advancement of pathological processes. Neuron-derived exosomes isolated from the peripheral blood of people with Down syndrome contained pathological tau protein. Such exosomes applied to mice by stereotaxic intracerebral injection contributed to the development of tau protein pathology in healthy animals and the formation of intracellular inclusions [62]. Similarly, in the case of studies on exosomes derived from cultures of human-induced neuronal cells from a patient with a genetic form of AD, it was observed that the administration of such exosomes to healthy mice resulted in increased phosphorylation of the tau protein and more inclusions occurring within hippocampal cells [63]. Moreover, it has also been shown that pathological forms of proteins transported by exosomes can significantly influence not only the initiation but also the propagation of the disease.
An interesting type of cargo carried by vesicles are various types of miRNAs. Their types and the target proteins that are subject to expression regulation are described in detail in the review by Thome et al. [64]. microRNAs, as endogenous, non-coding single-stranded RNA molecules, may play important roles in gene expression through post-transcriptional regulation [65, 66]. miRNAs are small particles – 20-24 nucleotides in length – and mainly bind to the 3’-untranslated region (3’UTR) of the messenger RNA (mRNA) that leads to gene silencing by mRNA cleavage, translational repression and deadenylation [65]. The role of miRNAs in the regulation of several physiological processes such as neuronal stem cell differentiation, neurogenesis, neuronal survival, and synapse formation is crucial in regulating the expression of different proteins. Various types of miRNAs may contribute significantly to the degeneration of the CNS and neuromuscular connections, resulting in the loss of motor control [67]. For instance, in AD miR-9, responsible for controlling BACE1 expression influences the APP cleavage; in PD miR-107 targeting progranulin transcript influences neuronal survival; in HD miR-128 binding to huntingtin and HIP1 transcripts regulates its expression and has an impact on neuronal differentiation; and in ALS miR-206 regulates the level of HDAC4 (histone deacetylase 4) altering chromosome structure and the expression of the other genes [68]. Therefore, the level of different miRNAs is often considered to be as biomarker of the progression of a disease.
THE IMPORTANCE AND APPLICATION OF EXOSOMES IN VARIOUS NEURODEGENERATIVE DISEASES
The use of exosomes as carriers for therapeutic substances in the treatment of CNS diseases requires their precise targeting of the appropriate cells. For this purpose, the exosome membrane is modified so that there is a polypeptide on its surface that will serve as a ligand and find the appropriate receptor on target cells. Modification of the exosome membrane before loading it with a therapeutic substance can be done using various methods, such as molecular cloning, the use of lentiviruses, attaching appropriate proteins to the exosome membrane, or through physical modifications such as the addition of magnetic particles [69]. Other modifications are presented in Table 2.
Table 2
Examples of modifications of exosome membranes in therapeutic trials carried out on animal models using vesicles to transport potentially therapeutic substances
Peptide/protein | Receptor | Target cells | Function | Ref. |
---|---|---|---|---|
Peptide C (RgdyK) | Integrin αvβ3 | Post-stroke brain area | Curcumin delivery and inhibition of the neuroinflammatory processes in injured area | [70] |
Peptide RGE | Neurokinin-1 | Glioma cells | Curcumin delivery and inhibition of the neuroinflammatory and apoptotic processes | [71] |
Peptide RVG | Lamp2b | Neurons, microglia, oligodendrocytes | siRNA providing and AD-related gene silencing | [54] |
Peptide RVG | Acetylocholine receptor | Neuronal cells | siRNA delivery and opioid receptor silencing to treat morphine addiction | [72] |
Alzheimer’s disease
AD is one of the most common degenerative diseases of the CNS with cognitive, neuropsychiatric and functional disturbances. AD, which is a late-onset disease, is also represented by early cases (< 50 years of age), which are most often genetically determined [73]. The hallmark of AD is the APP protein, which aggregates when improperly cut, creating characteristic deposits within and outside the cells of the nervous system. Such aggregates trigger a cascade of pathological processes leading to the death of cortical neurons in the brain. One of the first studies involving therapeutic trials involved packaging siRNA into exosomes which regulates the expression of the BACE1 enzyme, which cuts the APP protein into fragments that can transform into amyloid β [74]. In a mouse model of AD, such exosomes with siRNA were administered intravenously and a significant reduction in the level of BACE1 mRNA and protein itself was observed [54]. In another study using a mouse model of AD, exosomes containing miR-124a were administered to reduce apoptosis, resulting in an increase in the expression of the excitatory amino acid transporter protein (GLT1) on the surface of astrocytes, and through increased glutamate uptake an effect on synaptic activity was demonstrated [59]. In the same study, in the SOD1G93A mouse model of ALS, miR-124a packaged into exosomes was also administered intracerebrally and it was also shown that the level of the GLT1 protein responsible for communication between astrocytes and neurons increased significantly.
When exosomes enriched with miR-29, downregulating BACE1 and BIM expression, were administered intracerebrally to rats with induced AD, they improved the previously observed memory and learning deficits [75]. Exosomes derived from bone marrow mesenchymal cells were administered to mice with AD and were shown to improve the animals’ learning and memory abilities. At the same time, the levels of amyloid β, BACE1 and presenilin 1 were reduced, while the expression of neprilysin increased. Studies of exosomes enriched with a sphingosine kinase inhibitor and/or a sphingosine phosphatase receptor blocker allow the opening of a new therapeutic direction in AD [76].
The possibility of using exosomes to transport therapeutic substances is widely studied in relation to neurodegenerative diseases. Exosomes administered in a rat model of AD loaded with coenzyme Q10 improved cognitive abilities and reduced memory loss by affecting the levels of BDNF and SOX2 in the hippocampus [77]. Exosomes filled with curcumin were also used, inhibiting the phosphorylation of tau protein and preventing neuronal death in a mouse model of AD [78]. Comparing the effects of free quercetin and quercetin packed into exosomes, it was noticed that the exosomal system was much more efficient in penetrating brain tissue and improved cognitive functions more effectively in mice [79]. To date, only one clinical trial is registered in the clintrials.gov database involving human patients and concerns the administration of vesicles isolated from the culture of allogeneic cells collected from the patient (NCT04388982).
Parkinson’s disease
PD is a relatively common degenerative disease of the CNS that occurs as a result of the loss of dopaminergic neurons in the substantia nigra of the brain. The cause of PD may be acquired; however, some patients, especially when the disease develops early (< 40 years of age), may present with a genetic form of the disease. So far, > 20 genes have been identified in which mutations can cause the disease and show both autosomal dominant and recessive inheritance [80]. Clinical symptoms of PD include tremor and bradykinesia, gait disturbances, muscle stiffness and pain. Patients also experience mental symptoms – depression and anxiety. Attempts to treat PD using exosomes initially included intravenous administration of dopamine encapsulated in exosomes, which showed that this transport system is much more efficient than oral administration of dopamine, crosses the BBB and delivers dopamine to the brain (striatum and substantia nigra) and increases its content in the brain fifteenfold, while oral administration of dopamine in the most absorbable form achieves an absorption efficiency rate of only 1% [81]. Studies conducted in animal models of PD have shown that the use of vesicles derived from mesenchymal stem cells containing miRNAs whose levels are reduced in PD (e.g. miR-133b, has-miR-182-5p, has-miR-183-5p, has-miR-9, has-let-7) reduced the level of neuroinflammation, supported neurite growth, reduced the concentration of free radicals and prevented cells from entering the apoptotic pathway [82]. The registered and completed clinical trial NCT01860118 concerned the search for an exosome biomarker in patients with PD and tested whether administering the kinase inhibitor sumitinib to patients reduces the level of LLRK2 (a protein that is a product of a gene that is mutated in some forms of PD).
Amyotrophic lateral sclerosis
Amyotrophic lateral sclerosis (ALS) is a rapidly progressive neurodegenerative disease affecting the lower and upper motor neurons and spinocerebral pathways, causing muscle weakness, cramps, swallowing problems and ultimately respiratory failure. Usually, the cause appears to be idiopathic, but in some patients a genetic basis can be identified. Misfolded proteins and metabolic processes disorders are observed in cells: excessive oxidative stress, mitochondrial failure, neuroinflammation and calcium metabolism disorders [83]. Due to the ability of EVs to travel long distances in the body with body fluids, their potential role in transferring harmful substances from the brain to neuromuscular junctions and damaging their functions is considered. The cargo most frequently identified in vesicles from ALS patients were misfolded proteins: SOD1, FUS, TDP43 or toxic dipeptides that are the product of a dynamic mutation in the C9orf72 locus [84, 85]. When examining the SOD1-G93A mouse model, it was observed that mSOD1 protein accumulates in vacuoles of mitochondrial origin in cells even before the onset of disease symptoms, and then vesicles containing large amounts of mSOD1 are released. It seems that they initiate the signaling cascade leading to neuroinflammation, neurotoxicity and the spread of prion-like pathology, as well as inducing neuronal apoptosis [86]. These observations are also confirmed in the case of other forms of ALS and other proteins taking abnormal conformations. An example is the TDP-43 protein, the level of which in exosomes isolated from the plasma of ALS patients increases together with the level of neurofilament light chains. It has been noticed that normal U251 cells can acquire pathological properties as a result of incubation with exosomes isolated from the cerebrospinal fluid of ALS patients, and form structures resembling aggregates of the TDP-43 protein [87]. Proteins carried by vesicles that are not directly related to the cause of the disease belong to various groups of proteins involved, among other things, in the regulation of inflammatory processes (e.g. BLMH, CD163, FOXP3, CUEDC2, TNFR2), stress granule dynamics (e.g. CD177, SCAMP4), DNA repair and RNA metabolism (e.g. FUS, pFUS, TDP-43) [88, 89].
In addition to these proteins, vesicles originating from the cerebrospinal fluid of SOD1-G93A mice also contain pro-inflammatory cytokines: IL-1β, IFN-γ, and IL-6, which regulate the inflammatory reaction in response to toxic stimuli: protein aggregates, dipeptides produced due to dynamic mutation or oxidative stress [64].
Great hopes are associated with the use of EVs, especially those derived from stem cells, in ALS therapy. Examples of such therapeutic trials using animal models are presented in Table 3. Many studies have been conducted showing that exosomes isolated from adipose tissue stem cells, collected from mice representing the ALS SOD1-G93A model, have a neuroprotective effect on the nervous system [90].
Table 3
Results of therapeutic trials of exosome administration in animal models of amyotrophic lateral sclerosis
Exosome origin | Mechanism | Impact on biological function | Ref. |
---|---|---|---|
Murine ASC | – | Oxidative stress reduction | [91, 92] |
Murine ASC | Upregulation of antiapoptotic proteins Bcl-2α | Improvement in survival of neurons | [93] |
Murine ASC | Downregulation of proapoptotic proteins 9Bax caspase-3 | Improvement in survival of neurons | [93] |
Murine ASC | – | Decrease of activity of glial cells. Improvement in motor skills | [94] |
Human ASC | Phospforylation increase of CREB/CREB i PGC-1α | Reduction of cytoplasmatic aggregates. Improvement in mitochondrial functioning | [95] |
hBM-EPC | Decrease in apoptosis | Regeneration of brain endothelial cells | [96] |
hiASTR | Overexpression of miR-494-3p in vesicles isolated from induced astrocytes from patients with C9orf72 dynamic mutation – as a result of negative regulation of semaphorine 3A (SEMA3A) | Improvement in neuron survival and neurite network maintenance in vitro | [97] |
In a publication, currently available as a preprint, the authors provide information about the first clinical trial (phase I) of administering exosomes from Schwann cell cultures to patients by intravenous injection [98]. There are no registered clinical trials using exosomes.
Huntington’s disease
Huntington’s disease (HD) (OMIM #143100) is a rare autosomal dominant neurodegenerative disease. Clinically, it is characterized by progressive movement disorders: chorea, dystonia, eye movement disorders, and dysarthria, as well as cognitive disturbances. It is caused by a dynamic mutation causing an increase in the number of CAG microsatellite repeats in the HTT gene located on chromosome 4. The protein with the extended polyglutamine domain takes an abnormal conformation therefore aggregates containing mHTT appear in the cell nucleus [99]. In HD patients, it was observed that huntingtin incorrectly folded due to mutation, is carried in exosomes, which thus “infects” neighboring and more distant cells. Exosomes isolated from fibroblast cultures from HD patients and administered intraventricularly to newborn mice caused pathological changes in the latter [100].
In a mouse model of HD, exosomes containing miR-124, which appears to be a key mirR, were stereotaxically administered to the striatum. miR-24 is observed as having a reduced level of expression in HD patients. Although a reduction in the expression of the target gene encoding the RE1 transcription silencing factor (REST) was later found, there was no significant improvement in the clinical condition of the animals [101]. Perhaps the addition of other regulatory elements, such as miR-9, miR-22, miR-125b, miR-125b, miR-146a, miR-150, miR-240, could result in a greater therapeutic effect. In turn, the use of siRNA complementary to exon 1 of huntingtin by packaging it in exosomes and intravenously administering it to mice replicating the HD model resulted in a reduction in huntingtin expression and improvement of the animals’ motor skills [102]. There have been no registered clinical trials using exosomes to date.
THE FUTURE OF THE USE OF EXOSOMES IN THERAPY FOR DEGENERATIVE DISEASES OF THE CENTRAL NERVOUS SYSTEM
The use of vesicles in the treatment of degenerative diseases of the CNS can be implemented in many ways. Knowing their role in disease pathology and, in particular, their participation in the spread of pathological proteins, it seems that a natural strategy would be to (1) limit exosome biogenesis, (2) eliminate exosomes from the circulation, or (3) reduce vesicle uptake by target cells. Indeed, it has been shown that in the PD model the administration of various substances that reduce the expression of proteins related to the process of exosome biogenesis (e.g. siRNA lowering the expression level of ALIX, Slp4 and Slac2b proteins inhibiting Rab27a and b proteins) reduces their number and, consequently, reduces the number of α-synuclein aggregates and prevents the death of dopaminergic neurons [103, 104].
However, removing EVs from the circulation is less applicable in the case of neurodegenerative diseases, because the transport of abnormal proteins takes place primarily between the cells that built the CNS. However, in the case of cancer, antibodies against the surface antigens CD9 and CD63 can be used, which results in the increased phagocytosis of exosomes coated with such antibodies [105]. Reducing the uptake of exosomes by target cells can be achieved by using endocytosis inhibitors, e.g. cytochalasin D or destabilizing lipid rafts in cell membranes [106, 107].
A completely different therapeutic approach concerns the use of vesicles as packaging for medicinal substances. Since the blood-brain barrier is a major challenge in the diagnosis and treatment of degenerative diseases of the CNS, attempts to overcome this barrier, especially when delivering therapeutic substances to specific areas of the brain showing the presence of pathological processes, are currently targeted by researchers. Hence the development of so-called drug delivery systems, i.e. methods and technologies that increase the safety and effectiveness of administered therapeutics through better control of their level, duration of the activity and location in vivo. Such systems include liposomes, polymeric nanoparticles, solid lipid nanoparticles, nanogels and nano-emulsions that have been explored in recent decades. Some of these DDSs have already been used in clinical trials of neurological diseases, but their disadvantages may include, for example, high immunogenicity, toxicity [108], low stability [109] or low drug delivery efficiency [110]. In the case of the first observations of the use of EVs as DDS, it was noted that exosomes containing curcumin used in a mouse model of septic shock increased its anti- inflammatory properties [111]. Another example is exosomes containing miRNA, which, after intravenous administration to mice (subarachnoid hemorrhage model), effectively reached the brain [112, 113]. The key role in the effective use of EVs as DDS is their packaging with appropriate cargo and the choice of the route of DDS delivery to the target organ, which can be complicated, especially in the case of the brain.
The step of loading the vesicles with a substance suitable for therapeutic purposes is currently based on the use of several platforms: incubation, electroporation, the use of ultrasound, extrusion of the vesicles from the cells, the use of thawing and freezing cycles and the use of saponins [111, 114-118]. Protocols for the production of drug-filled vesicles are adapted to the source of the vesicles, the method of their isolation, the type of substance that is planned to be packaged and the subsequent route of administration to the body. It was shown, for example, that the use of transfection and electroporation of vesicles to pack siRNA molecules into them resulted in damage to the vesicles, precipitation of siRNA on membranes and ultimately loss of cargo [119]. Meanwhile, the electroporation packaging of miR-31-5p into exosomes isolated from milk was found to be resistant to degradation and achieved good cellular uptake efficiency [120]. However, it is generally believed that the use of electroporation to package siRNA leads to its aggregation and causes the retention level in vesicles to be < 0.05% [121]. Effective cargo packaging increases therapeutic options while reducing the potential toxic effect of the drug. Nevertheless, current vesicle uploading protocols only achieve 30% efficiency compared to synthetic carriers [121].
The therapeutically loaded EVs should be kept in good condition until application and subsequent transport in the body. To achieve this, it may be necessary to modify the membrane of vesicles to increase their biodistribution, half-life, and ability to find target cells, which together contribute to their therapeutic potential. Storing vesicles in improper conditions may result in changes in their amount and properties [122, 123].
The route of administration of vesicles is also essential to maintain their therapeutic potential. Currently, the leading methods of introducing vesicles into the body for the treatment of neurodegenerative diseases are the intravenous route, oral or intranasal administration and stereotactic injection. The first three methods are quite well tolerated and accepted, while intracerebral injection raises a lot of resistance due to its invasive nature. This route of administration in a mouse model of AD improved learning and memory processes, reduced deposits and levels of amyloid beta, and normalized the level of inflammatory cytokines [124]. Many similar studies have been carried out on animal models and involved the administration of vesicles extracted from milk and filled with curcumin, as well as vesicles of plant origin, and these studies are of great importance for future therapeutic applications in humans [125-128]. The oral route of administration seems to be the least effective due to the action of digestive enzymes and the difficulty in getting vesicles to reach the brain; the intranasal route has its limitations due to the small surface area of olfactory cells, which implies the administration of only small doses; and in the case of the intravenous administration of the vesicles we encounter their short half-life, resulting mainly from elimination by macrophages.
To eliminate possible limitations, various strategies are used to modify vesicles, mainly involving the modification of their membrane: (1) targeting of exosomes by proteins on their surface, (2) exosomes obtained artificially, (3) modification of target proteins, (4) exosomes modified by protein receptors, (5) physically and chemically modified exosomes.
SUMMARY
In numerous studies of exosomes, which are one of the types of EVs most often used in research, over 4,000 proteins and 2,400 types of RNA have been identified.
Vesicles that carry biological substances in the body perform 3 basic functions: (1) participation in the pathological mechanism of a given disease, (2) the role of a biomarker that has both diagnostic and prognostic functions, and (3) a role in therapeutic activities, especially: vesicles as a potential therapeutic target that should be eliminated, and vesicles as a therapeutic agent carrying desired therapeutic substances to appropriate regions of the body including the brain.
Research on EVs in the context of neurodegenerative diseases has been going on for many years, but their practical use is currently limited to studies on animal and cellular models. Despite optimistic forecasts and seemingly encouraging results of experimental research, practical application in the form of clinical trials in neurodegenerative diseases is so far extremely rare.